Ask Ethan: Do protons really contain charm quarks?
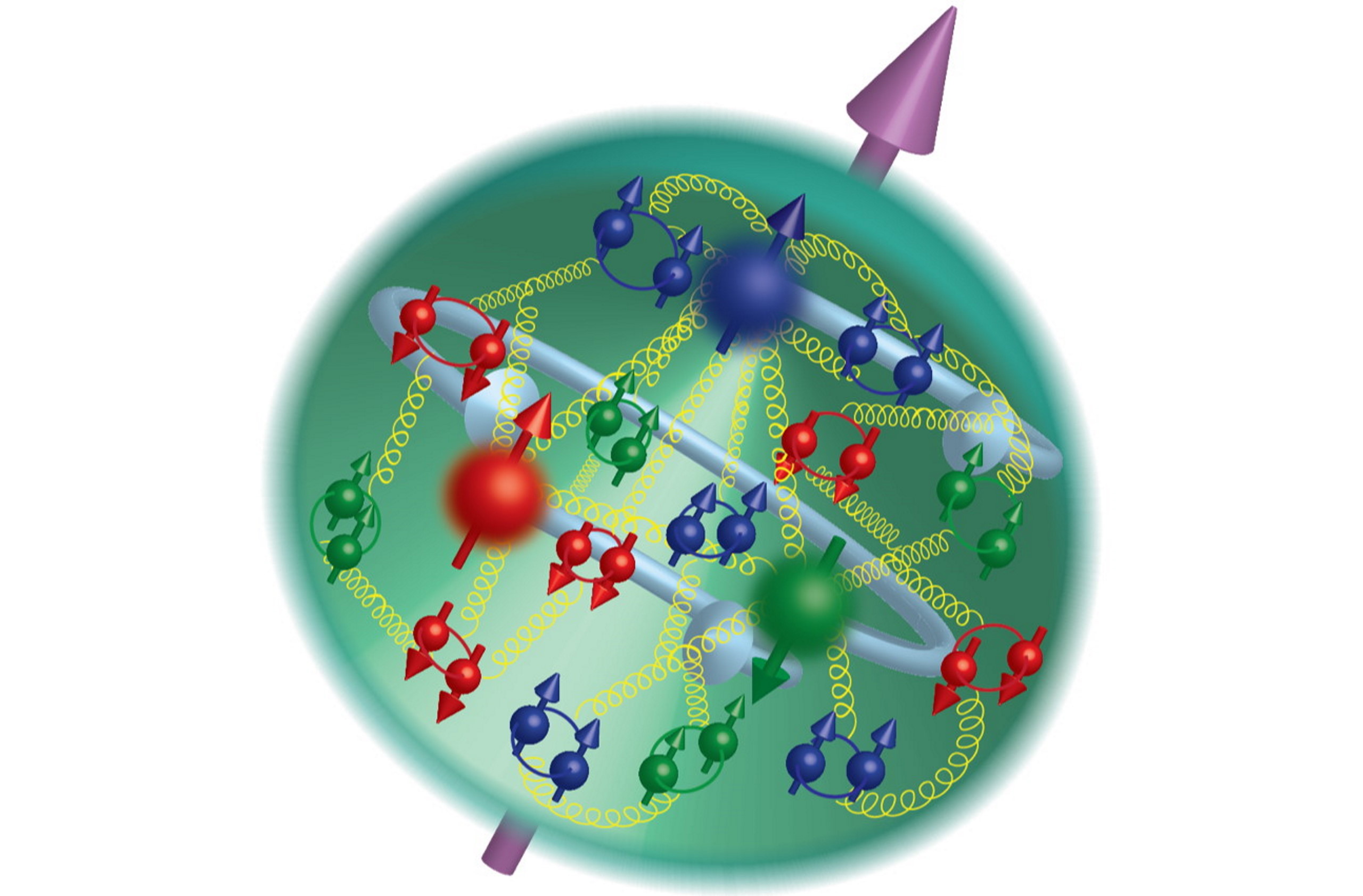
- Protons are composite particles, made up of quarks and gluons inside, which we can probe and detect through particle physics experiments and techniques like deep inelastic scattering.
- By measuring what comes out of a high-energy collision, we can reconstruct what happened back at the collision point, determining which constituent particle(s) inside the proton collided.
- Instead of just up and down quarks (as well as gluons), however, we recently found a charm quark inside the proton from a collision. How is that possible?
At the start of the 20th century, we were still figuring out what the structure of matter was. We knew everything was made up of atoms, and that there were negatively charged electrons within them, but the rest of the atom was a mystery. Over the course of the past 120 years, we subsequently learned that there was a small, massive, positively charged nucleus anchoring every atom. That nucleus itself is composed of nucleons — protons and neutrons — with each one itself made up of quarks and gluons. Protons consist of two up and one down quark apiece, while neutrons are made of two down and one up quark.
But there are four other fundamental types of quark: strange, charm, bottom, and top, with the latter three all heavier than the proton itself. How would it be possible, then, for such a particle to be found inside a proton? That’s what our Patreon supporter Aaron Weiss wants to know, asking:
“[H]ow there can be charm quarks in protons? I thought charm quarks were more massive than protons, so how is this possible? What does it mean that ‘heavy quarks also exist as a part of the proton wavefunction’ [as stated in this paper]?”
It’s a deep question that makes us fundamentally reconsider how matter behaves on the tiniest scales. Let’s dive in!
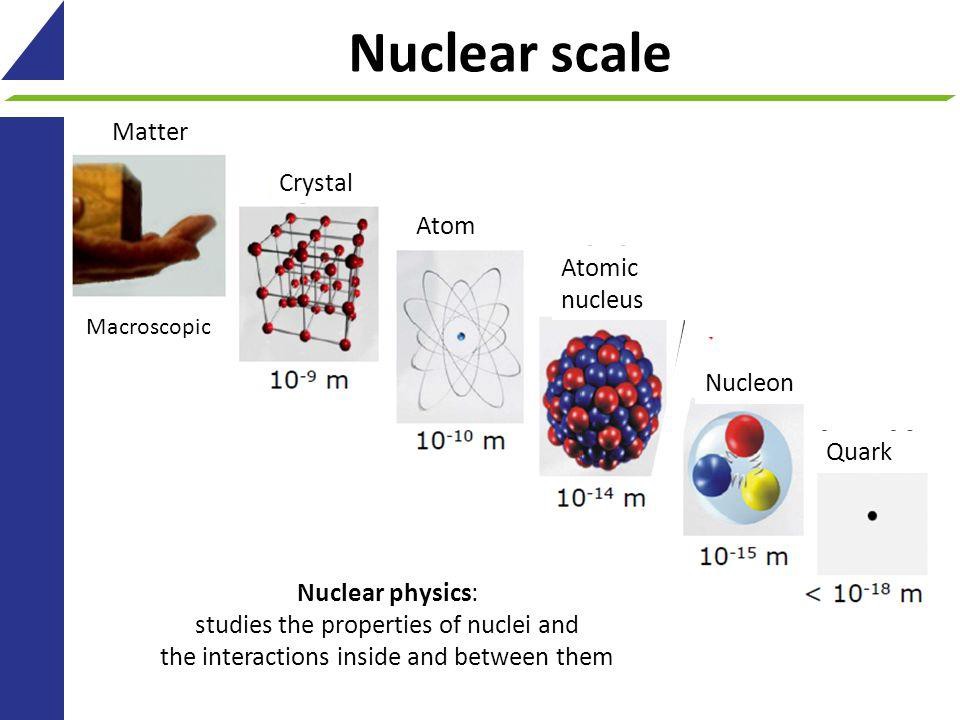
At an elementary level, we understand that everything that exists in the Universe is composed of fundamental, indivisible quanta: particles that obey the bizarre and often counterintuitive rules of quantum physics. The normal matter we’re familiar with is made out of atoms, which themselves are made out of nuclei and electrons, with nuclei composed of protons and neutrons, each of which has their own unique internal structure.
When most of us think about the internal structure of a proton or neutron, we think about the three quarks that determine their properties like electric charge, their magnetic moments, their masses, and more. The lightest particles are always the most stable, as heavier particles can decay to lighter ones; hence it’s no surprise that the normal matter we’re familiar with is made of the lightest two quarks: up and down.
With up quarks having a charge of +⅔ apiece and down quarks possessing charges of -⅓ each, the way you arrive at a proton (with a charge of +1) is to combine two up quarks with one down quark (since ⅔ + ⅔ + -⅓ = +1), while the way you get a neutron (with a charge of 0) is to combine two down quarks with one up quark (since -⅓ + -⅓ + ⅔ = 0).
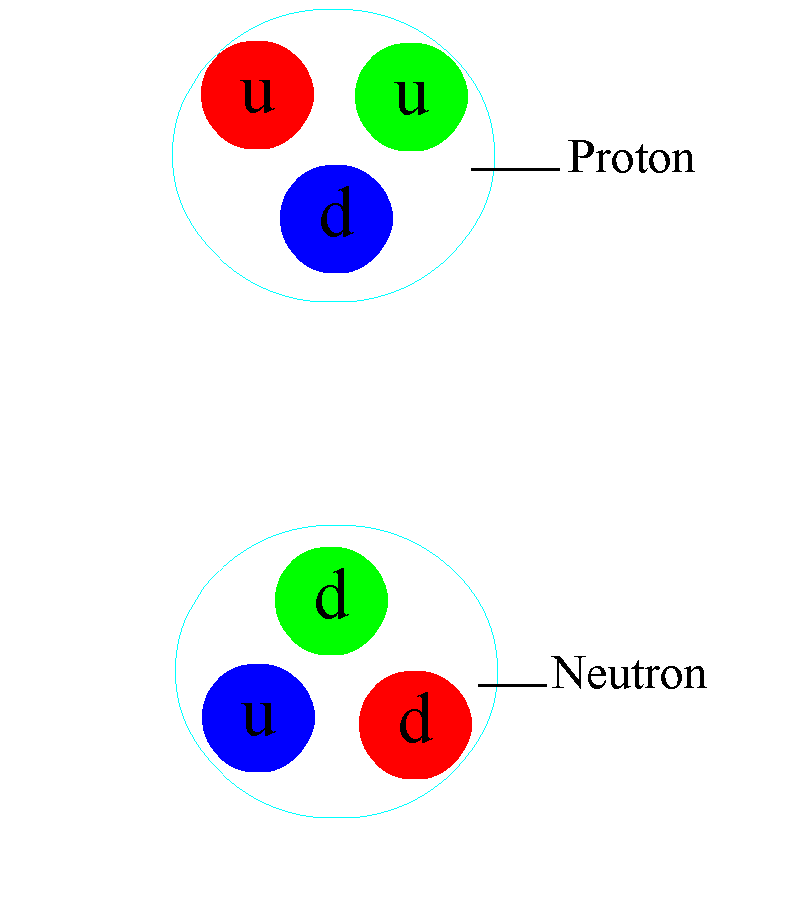
The reason you need three quarks is because of the way the strong force works. The strong force is what allows quarks to form bound states, and obeys the rules of a theory known as quantum chromodynamics. In chromodynamics, each quark has a “color charge” to it, which each gluon has a “color-anticolor” combination assigned to it. Colors can be red, green, and blue, while anticolors are their opposing colors on the color wheel: cyan, magenta, and yellow. The only stable, bound states that are allowed to exist, however, are combinations that are completely colorless as a whole.
When each color is paired with its respective anticolor, it makes a colorless combination; when all three colors or all three anticolors are combined, they also make a colorless combination. As a result, only combinations of:
- three quarks,
- three antiquarks,
- a quark-antiquark pair,
- or combinations of two or more of the above,
are admissible as bound states. The up and down quarks are very light, but since they’re bound together by the exchange of gluons, the entire mass of the bound state (e.g., a proton or neutron) can be quite large. Binding energy is just as much a form of energy as rest-mass energy, and they all contribute to the mass of a nucleon.
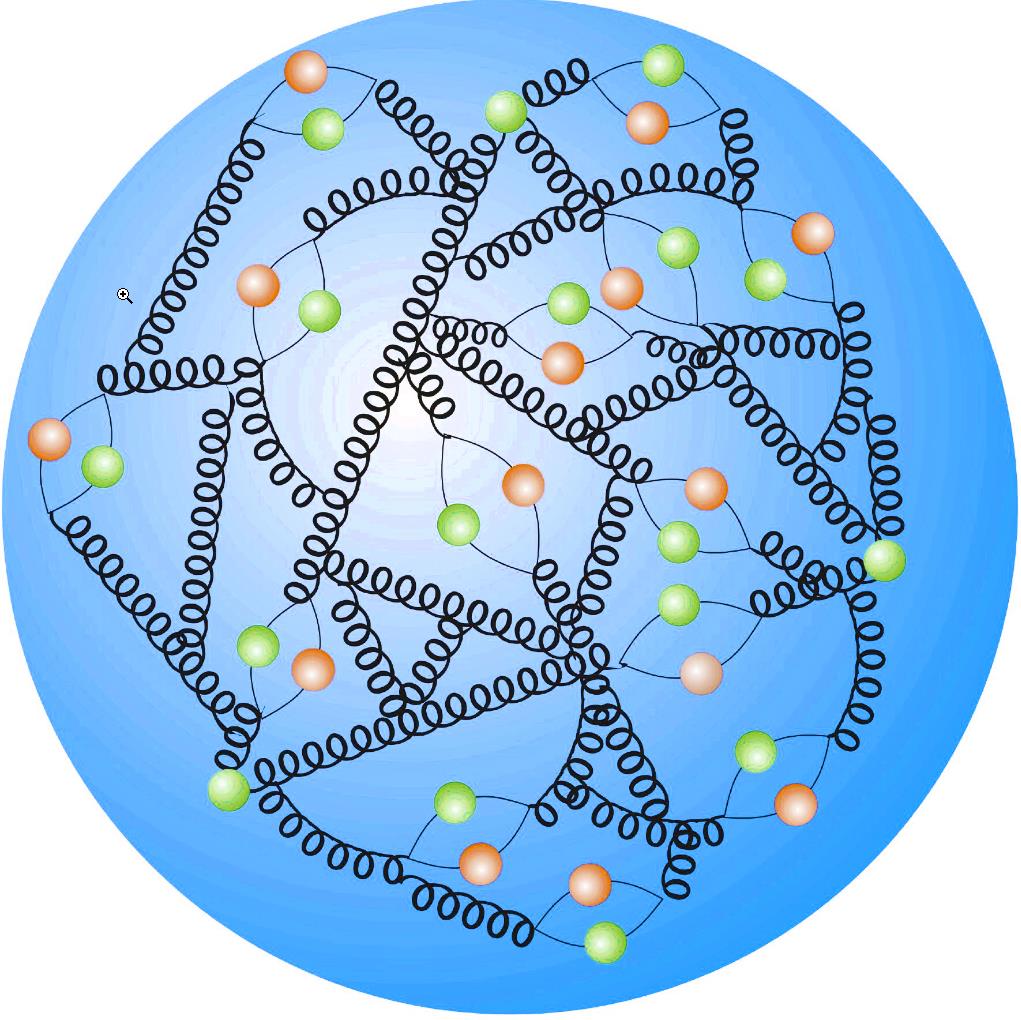
But then, we need to ask about the internal structure of something like a proton. The way you probe that is by firing other particles at it: other protons, photons, or electrons, for example. The electron is perhaps the most pristine way to probe the internal structure of a proton, because:
- it’s a fundamental, point particle, not a composite particle,
- it has an electric charge, like quarks, but not a color charge, so it can’t directly interact with gluons,
- the post-collisional debris that comes out of an electron-quark collision can be reconstructed in experimental particle physics,
- and the physics of electron-quark interactions can be theoretically calculated in a straightforward fashion within the Standard Model.
Additionally, as we’ve gone to higher and higher energies in our collisions, we’ve gotten to see and notice different effects. Higher energies correspond to shorter timescales and distances for interactions, allowing us to get more and more granular when determining the internal structure of something like the proton.
It’s been precisely through experiments that leverage these factors that we’ve revised our picture of what’s going on inside the proton over the past ~40 years or so, and how we’ve just very recently found that yes: from a deep inelastic scattering experiment, there sometimes really are particles that “shouldn’t be there,” like charm quarks, within the proton.
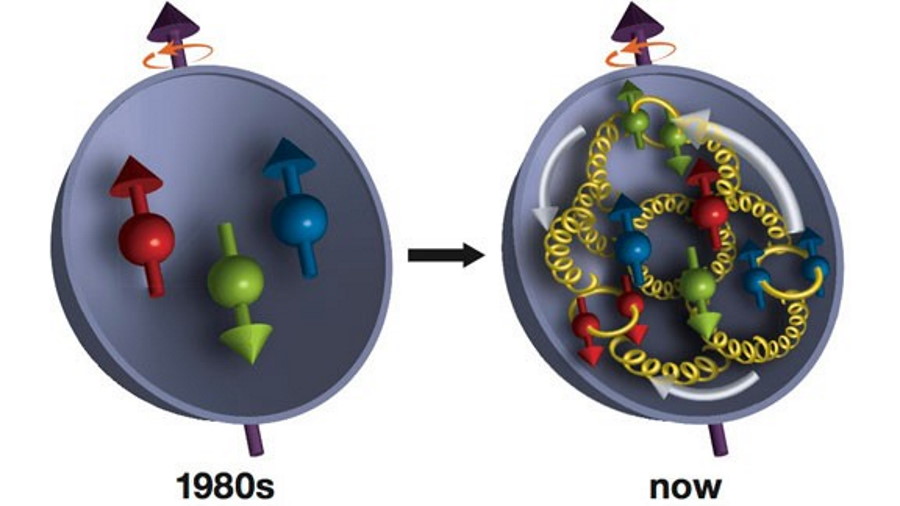
At low enough energies, all you see from smashing things into protons and neutrons are the whole nuclei themselves. Quarks weren’t discovered until the second half of the 20th century for the simple reason that we weren’t hitting protons and neutrons into one another (or with other particles) with enough energy to reveal their internal structure.
As you increase the energy, however, new phenomena start to appear concerning the internal structure of these particles. The first thing you’re able to detect about the internal structure of the proton is the three valence quarks: the two up and one down quark that give the proton its macroscopic properties. Collide two protons at these energies, and practically 100% of the collisions that occur can be successfully modeled as quark-quark collisions between one of the three valence quarks in each proton.
But if you go to still higher energies, you start to find an even deeper, more complex structure inside the proton. In particular, you first start noticing that there are gluons inside the proton, with quark-gluon collisions and eventually gluon-gluon collisions becoming the most common and important type of interaction that occurs when you smash two protons together.
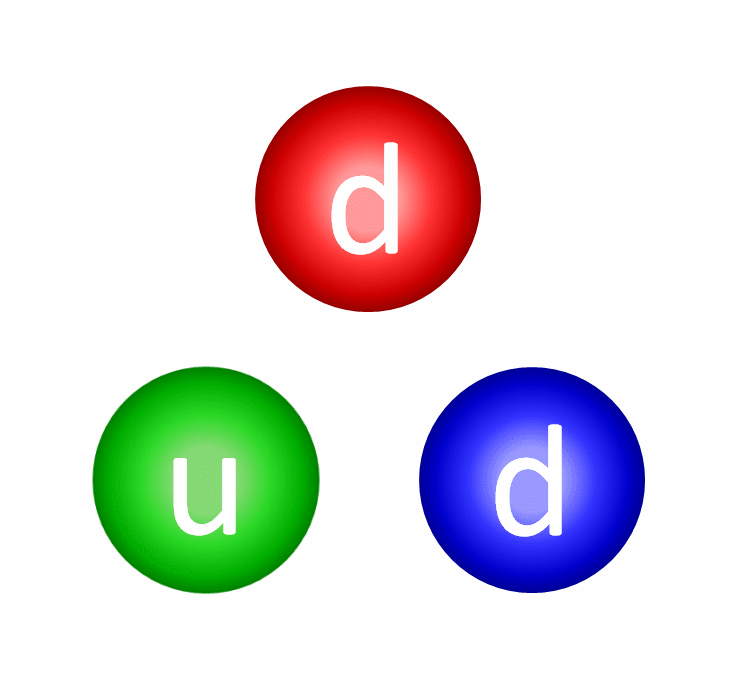
Despite what you might think, it isn’t only the valence quarks that contribute to the probability of having quarks collide within the proton; there’s a phenomenon known as “sea quarks” as well. Whenever you have a gluon being exchanged within the proton, there’s a finite, non-zero probability that the gluon will spontaneously:
- convert into a quark-antiquark pair,
- propagate through the internal space between the valence quarks in the proton,
- recombine into a gluon,
- and then finish the exchange with the other valence quark.
We might more commonly think of Heisenberg’s uncertainty principle as applying to empty space: where particle-antiparticle pairs can pop in-and-out of existence from the quantum vacuum, so long as the amount of time they exist for obeys the energy-time uncertainty relation.
But part of what comes along with our quantum understanding of the Universe is that every quantum has a finite, non-zero change of experiencing what we call radiative corrections and loops: where a particle can either give off a boson or can have a boson split into a particle-antiparticle pair before recombining. At low energies, and/or with small numbers of collisions, we’re unlikely to see such an event. But if you add up large numbers of high-energy events, evidence for these interactions will begin to accumulate.
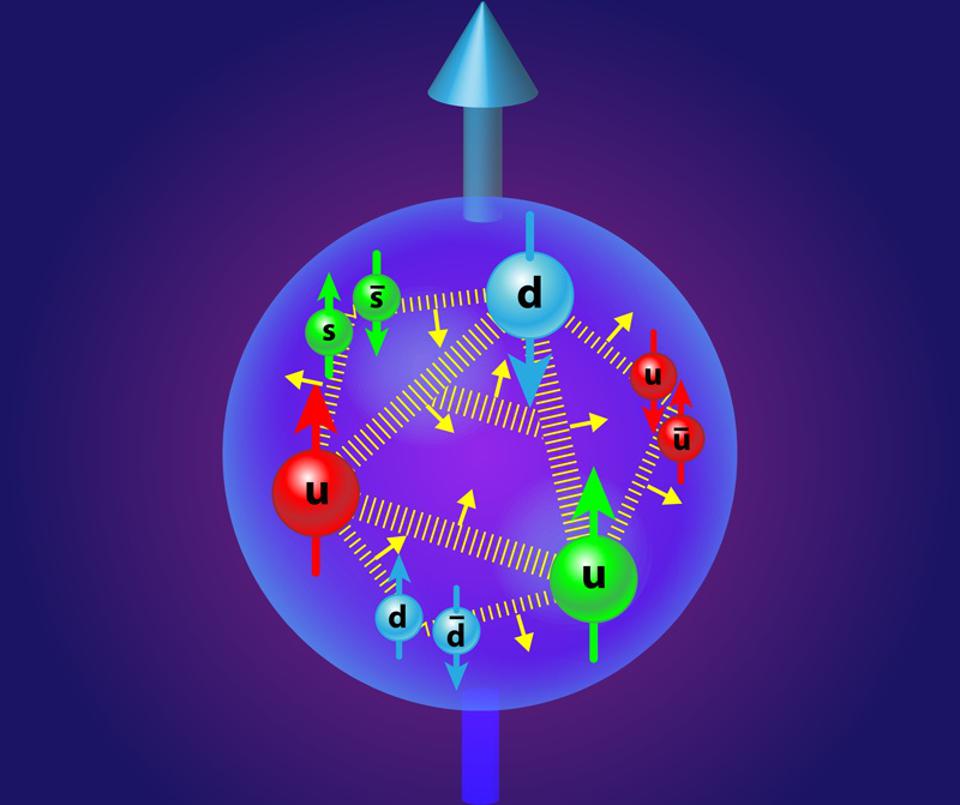
Now, gluons — the particles that do this “splitting” into particle-antiparticle (quark-antiquark) pairs inside the proton — are massless, but they’re not energy-less. In fact, the binding energy of the three valence quarks is what’s responsible for some ~98+% of the proton’s mass, and that energy is distributed among all the constituents of the proton: the valence quarks, the gluons, and by extension, the sea quarks as well.
Most of the time, the sea quarks (and antiquarks) are simply pairs of up-and-down quarks (and antiquarks), because those are the lowest rest mass quarks (and antiquarks) of all, containing less than 1% of the proton’s mass apiece. The strange quark (and antiquark), the third lightest of the quarks, is much heavier: it has about 10% of the mass of the proton, which means that a strange quark-antiquark pair makes up 20% of the proton’s mass.
With enough energy available, remember, it should always be possible to create particle-antiparticle pairs via Einstein’s most famous equation: E = mc². It shouldn’t surprise anyone that, among the sea quarks created by strong force interactions inside the proton, sometimes strange quarks (and antiquarks) are in there among the more common ups-and-downs.

But it’s maybe much more surprising to find, as an August study indicated, that charm quarks are in there as well. After all, the charm quark — the fourth lightest among the quarks — has a mass that’s about 136% the mass of the proton. It should be energetically forbidden for a gluon, which never has more than a portion of the total energy of a proton, to split into a charm-anticharm pair; there simply isn’t enough energy available from E = mc² to make it happen.
It turns out, however, that isn’t the dealbreaker you might expect it to be. When we energetically probe the interior of a proton, we find that there is indeed a sea of internal particles, but there’s no limit to how deep and dense this sea goes. The more energetically you interact with a proton — and, remember, that high energy corresponds to short wavelengths, short distances, and short timescales — the denser this sea of internal particles appears to be.
But even if such an interaction reveals that a charm quark exists, that doesn’t necessarily mean that we’re finding a charm quark that’s intrinsically part of a proton. We have to be careful that, when we detect a particle inside a proton, it’s not being detected as a result of the aftermath of an energetic interaction, but rather as a result of a particle being intrinsic to a proton itself.
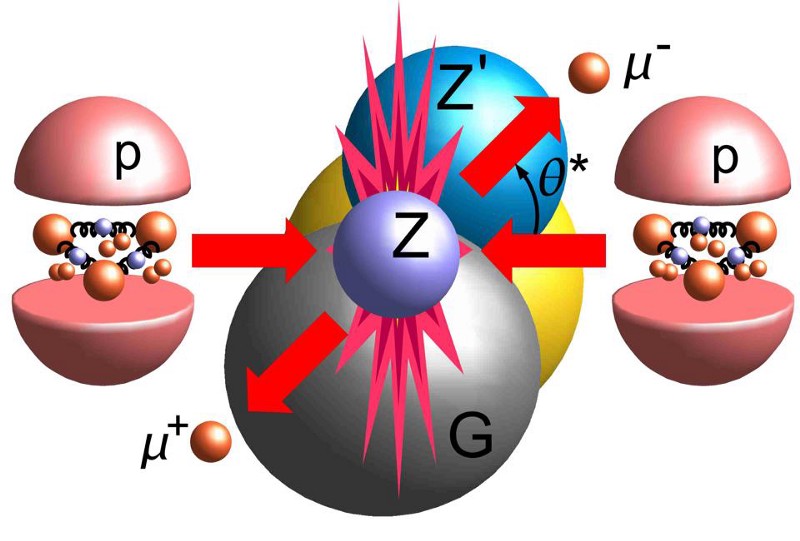
As long as the charm-anticharm pairs produced are virtual (i.e., as a result of a gluon spending some of its time as a quark-antiquark pair), this shouldn’t surprise us. In fact, looking at very small-scale, short-time interactions allows us, via Heisenberg’s uncertainty principle, to temporarily borrow some extra energy from the energy-time uncertainty relation. As long as that extra energy allows the creation of a charm-anticharm pair — or, for that matter, a bottom-antibottom and/or a top-antitop pair — they should exist. In fact, from the physics of quantum chromodynamics, we’re certain that if we were to somehow alter the masses of either the bottom or top quark, the mass of the proton would shift in response.
But this particular claim is different, and despite being published in the journal Nature, isn’t as sure of a slam dunk as we’d want. It’s a claim that the charm field we’re detecting is something extra: in addition to the charm field that should exist from these perturbative QCD effects that create the sea quarks. In other words, they’re claiming to find that there’s some “extra charm” in the proton arising from the valence quarks and gluons. And that claim, well, it all hinges on a combination of aggregate data, machine learning, models for the distribution function of the quarks inside, and the robustness, of, well, I’ll let you see the critical figure from the paper for yourself, below.
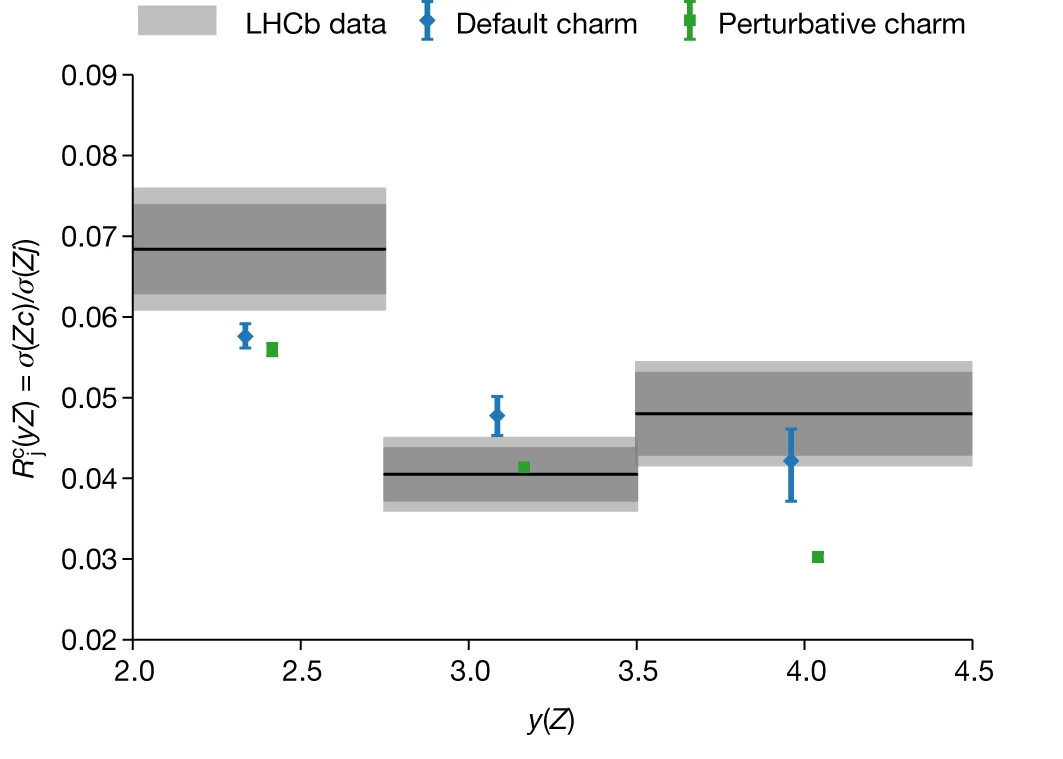
The claim that there’s “more charm quark in the proton” than what you’d expect from this virtual pair production rests on the blue points, above, being a better fit to the data than the green points.
Is it?
Yes. But not by the five-sigma significance normally required to announce a discovery in particle physics; it’s about a three-sigma effect, or something with a still-substantial chance of being a fluke. In fact, in particle physics, most three-sigma effects that are detected do turn out to be flukes, rather than new discoveries. Whether this turns out to be real or a fluke it worth further investigation, but it shouldn’t be taken as a given that the proton is intrinsically “extra charmy” just yet.
This is a very difficult problem, because we’re talking about virtual particles in a theory that’s very hard to accurately compute certain quantities. Virtual particles aren’t bound by the hard-and-fast rules of real particles: they have intrinsically uncertain properties, including mass and energy. Whereas a “real” charm quark always has a specific mass that’s 136% times greater than a proton, these virtual charm quarks arising from the gluons can take on any mass, including even negative values!
The cool part about this claim is that we’re actually closing in on being able to measure the contributions of the quarks, inside the proton, that arise from the gluon field due to quantum chromodynamics. It’s possible — and the early indications are that it might actually be so — that there’s more to the proton than we’ve assumed thus far. But, as is so often the case, it will take more and better data, and a better understanding of physics at the smallest, highest-energy scales, to be sure!
Send in your Ask Ethan questions to startswithabang at gmail dot com!