Gravitational Waves Win 2017 Nobel Prize In Physics, The Ultimate Fusion Of Theory And Experiment
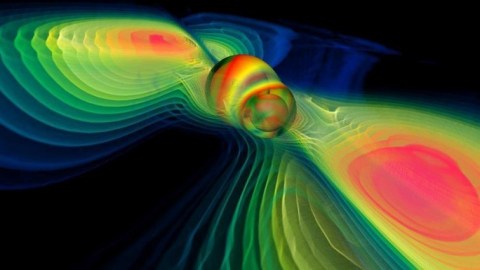
A well-deserved award for the discovery over a century in the making.
“Well, I walked into Building 20 and looked in at the various little labs. There was a bunch of people doing something that looked to me to be sort of interesting, and since I knew all this electronics, I asked them, “Look, can you use a guy?” And I sold myself off as a technician for about two years.”
–Rai Weiss, on the start of his physics career at MIT
In a moment over 100 years in the making, the 2017 Nobel Prize in physics was just awarded to Rainer Weiss (1/2), Kip Thorne (1/4), and Barry Barish (1/4), for pioneering work in the discovery of gravitational waves. Weiss, an experimentalist who first conceived of using interferometry for this purpose, Thorne, a theorist who helped tease out the signals that various astrophysical phenomena would produce, and Barish, a master of instrumentation who led LIGO during its crucial developments in the 1990s and beyond, are certainly among the most deserving of this prize. However, they were just three of a great number of people involved in the planning, construction, and the formation of the LIGO collaboration, which in 2015 directly detected the ripples from a gravitational wave for the first time. While all the glory is going to the 1,000+ members of the LIGO collaboration over its 40+ year history, the story of experimentally detecting gravitational waves goes back much farther. The 2017 Nobel Prize is a culmination of theoretical and experimental work dating all the way back to Einstein.
When General Relativity first came on the scene, it brought forth a new way of looking at the Universe: with matter and energy existing in the fabric of spacetime. Matter and energy told spacetime how to curve; the curved spacetime in turn told matter and energy how to move. A number of consequences arising from this new theory were soon after derived, including the existence of black holes, the fact that masses acted like a gravitational lens, the need for an expanding or contracting Universe, and the existence of a new type of radiation: gravitational radiation. When a massive particle moved through space where the curvature changed from one point to the next, it had no choice but to emit gravitational waves to conserve energy and momentum. The details begged to be worked out.
Einstein himself first predicted gravitational waves as a consequence of his theory, then backtracked and convinced himself they couldn’t exist. After 20 years of changing his mind back-and-forth, he wrote a paper in the 1930s with Nathan Rosen, convinced that gravitational waves were mere mathematical artifacts of General Relativity. The paper was rejected from Physical Review, as referee Howard Robertson, one of the four scientists for whom relativity’s expanding Universe solution is named, had found critical errors in their work. The argument continued into the 1950s, with Rosen contending that gravitational waves could carry no energy, and therefore weren’t physical. But Felix Pirani, Richard Feynman, and Hermann Bondi proved that they did. The key now was to predict and detect them.
On the theoretical side, it became clear what properties gravitational waves had. How they propagated, alternately compressing and expanding space in perpendicular directions, and how much energy they carried. The strongest waves were generated by the largest masses undergoing the most rapid motion through the most strongly-curved spacetimes: in the vicinity of collapsed objects like white dwarfs, neutron stars, and black holes. Developments in numerical relativity, including in perturbative expansions to Newton’s laws that incorporated these strong-field effects, allowed scientists to calculate which systems would produce gravitational waves, and to what extent. With the development of ultra-powerful computers, templates for predicting the waveforms of gravitational waves abounded, and became more and more precise.
On the experimental end, Joseph Weber was the first to pioneer a system for detecting gravitational waves: a series of resonant bars that were placed in a vacuum and were extremely sensitive to any gravitational waves of a particular frequency that traveled through space. Although Weber claimed detections beginning in the 1960s, his results could not be reproduced, matching with theory that predicted waves far out of the range of what his bars would be sensitive to. On the other hand, indirect evidence for gravitational waves came instead from pulsars — rapidly rotating neutron stars — that orbited other neutron stars. As these two compact masses swirled around each other, their periods decayed: evidence that energy was being carried away. Where did that energy go? It had to be gravitational waves.
Russell Hulse and Joseph Taylor won the Nobel Prize 24 years ago for their work on the first binary pulsar, which itself was done in the 1960s and 1970s. Back in the 1970s, also, was where the idea for LIGO came from. Sure, space would expand in one dimension while contracting in a perpendicular one, oscillating back-and-forth, so long as a gravitational wave passed through them. Rai Weiss was the one who first conceived of the idea of using an interferometer to make the detection, and made incredible contributions in the early design and instrumentation technique; Weiss receives half of the prize this year.
Thorne was a theoretical advocate and one of the pioneers in the numerical work that allowed various systems — like the inspiraling and merging black holes that LIGO finally saw — to be predicted. Without such outstandingly precise predictions about what signals each system should produce, it would be impossible to know what signal should be sought for amidst the noise. Barry Barish, meanwhile, was a master builder of the gravitational wave detectors, and a driving force behind transforming LIGO from an idea into the incredible set of observatories that it is today. He took over the project in 1994, resurrected the floundering idea, and transformed it into a set of detectors so impressive they could detect the merger of black holes more than a billion light years away, something that’s been accomplished four times now. Thorne and Barish share the other half of the Nobel award.
The detection of gravitational waves is not only surely worth a Nobel Prize, but has transformed our idea of what’s possible in astronomy. Multiple detectors, set up around the world, can pinpoint the location of a source; can detect the time-delays between detectors, confirming the speed of gravity equals the speed of light; can measure the orientation/polarization of the signals, and much more. Black holes will be detected, in the future, down to lower and lower masses, as gravitational wave astronomy becomes more and more precise, and more detectors come online. And eventually, even neutron stars and other light-producing sources will have their waves directly detected, ushering in an era where gravitational wave and traditional, telescope-based astronomy overlap.
The 2017 Nobel Prize in Physics may have gone to three individuals who made an outstanding contribution to the scientific enterprise, but it’s a story about so much more than that. It’s about all the men and women over more than 100 years who’ve contributed, theoretically and experimentally and observationally, to our understanding of the precise workings of the Universe. Science is much more than a method; it’s the accumulated knowledge of the entire human enterprise, gathered and synthesized together for the betterment of everyone. While the most prestigious award has now gone to gravitational waves, the science of this phenomenon is only in its earliest stages. The best is yet to come.
Ethan Siegel is the author of Beyond the Galaxy and Treknology. You can pre-order his third book, currently in development: the Encyclopaedia Cosmologica.