Ask Ethan: Do my atoms really “touch” each other?
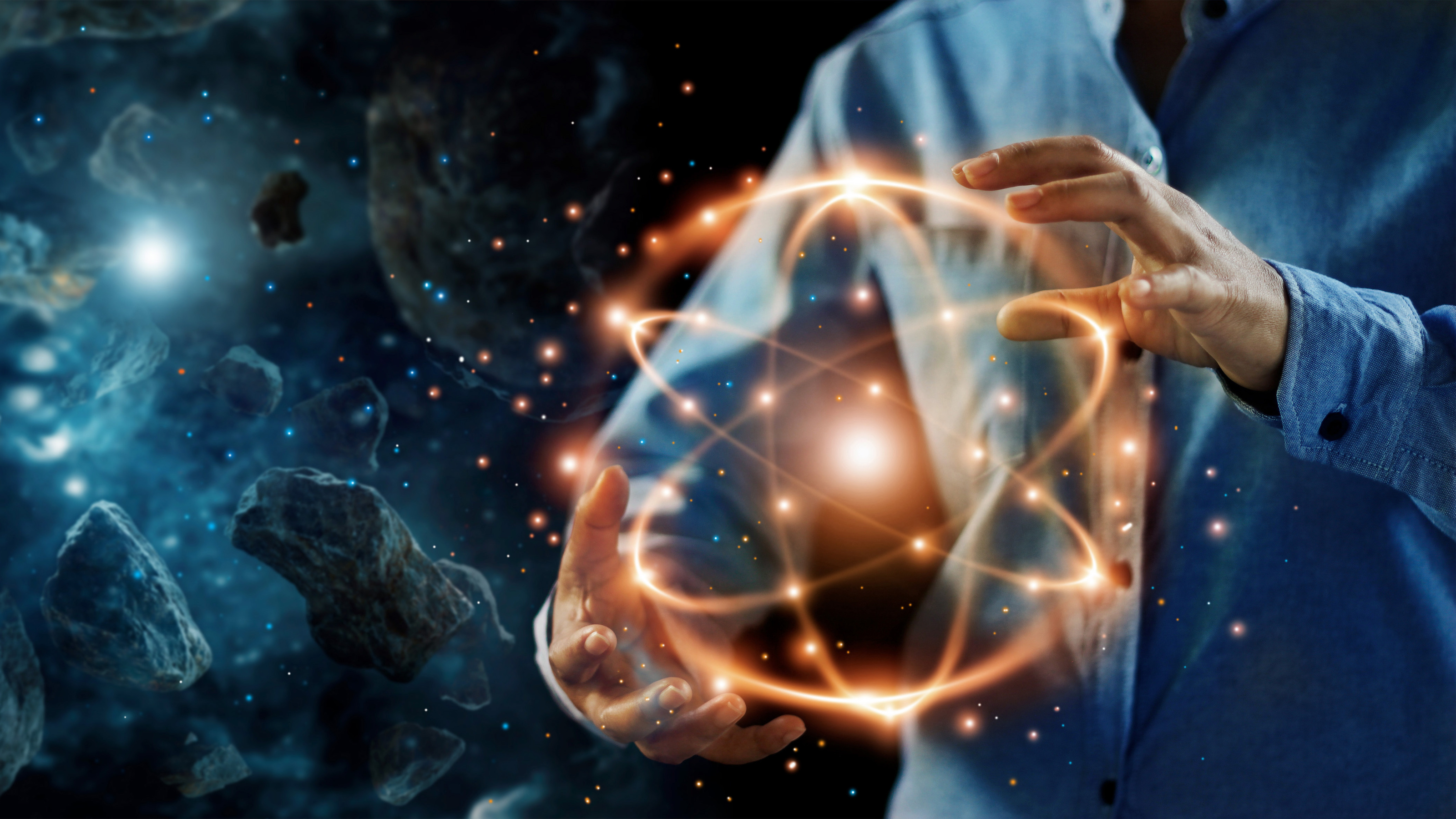
- The sensation of touch allows you to experience what other objects are actually like, as your atoms experience sensations from the experience.
- But when you bring objects close to one another, and even when you feel them touching, they’re clearly not bonded together, so what is it that you’re feeling?
- Contrary to what you might expect, the sensation of touch doesn’t actually involve two atoms in contact with each other. The physics of “touching” is more complicated than you realize.
One of the most counterintuitive things about existence is the notion of forces. In order to experience a force — that is, to feel the influence of something else on us — two objects don’t necessarily even need to touch, or be in contact, with one another. Objects on Earth’s surface feel Earth’s gravity, but so do airplanes, satellites, and even the Moon. An electrically charged object attracts and repels other electrical charges, regardless of how far away they are from each other. And, in a more familiar fashion, two magnets flipped so that their north poles face each other mutually repel one another so strongly that even the strongest humans cannot bring them completely together.
So what happens, then, when you try to bring your thumb and forefinger together? How close do they actually get, and do they ever truly “touch” one another? That’s what Peter Mead wants to know, writing in to ask:
“When I hold both hands out in front of me with my two forefingers pointed toward each other then bring them together, the space between my fingers gets smaller and smaller. I can see and hold them a bit less than a millimeter apart before they touch. Is there an instant, just before they touch, where my fingers are only an atom’s (or sub-atomic) width apart? Or does space somehow behave differently on such a small scale?”
Clearly, there’s a big range in between what we can see (a little under a millimeter) and the size of an atom (about one ten-billionth of a meter). Let’s find out what happens on those tiny scales.
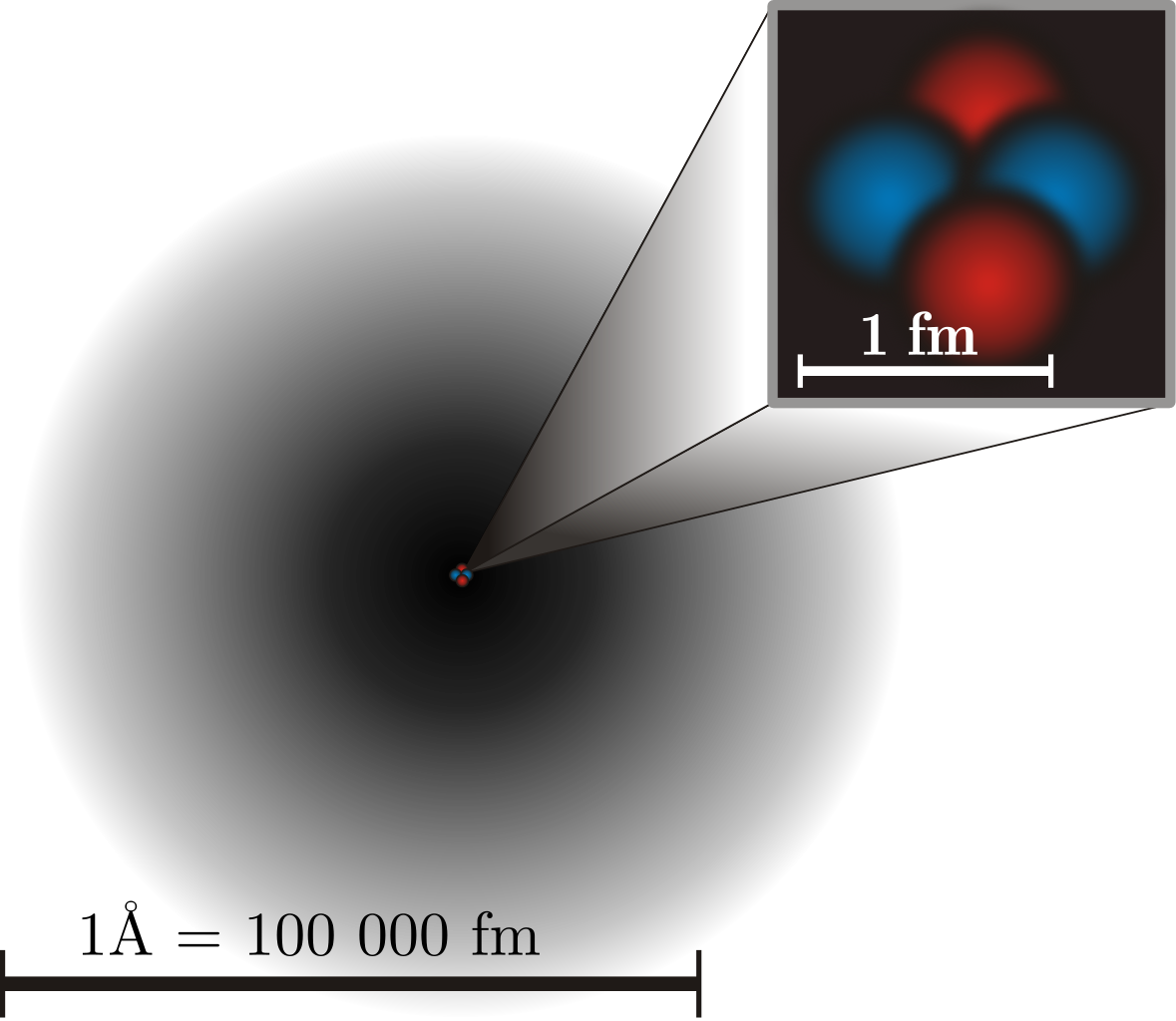
Although we’re going to go down to very small scales to address this question in full, it’s important to recognize that “small” doesn’t necessarily mean “quantum” the way you might intuit. Yes, quantum effects typically rear their heads in isolated, single-or-few particle systems and tend to disappear if there are many particles interacting frequently, which is a hallmark of (most) macroscopic phenomena. However, while quantum effects normally appear on atomic scales or below, the more classical effects — including gravitational and electromagnetic effects — can never be ignored, and frequently dominate even over inherently quantum ones, even on the smallest scales of all.
Therefore, the first step is to recognize that your body is made of atoms, and that while the atoms within your fingers are bound together into molecules that comprise the organelles that make up your cells, it’s still fundamentally all atoms: electrons orbiting atomic nuclei. Even though it’s a long way down from the macroscopic world (fingers) to the atoms and the subatomic particles that make even the atoms up, that’s what the structure of matter truly looks like.
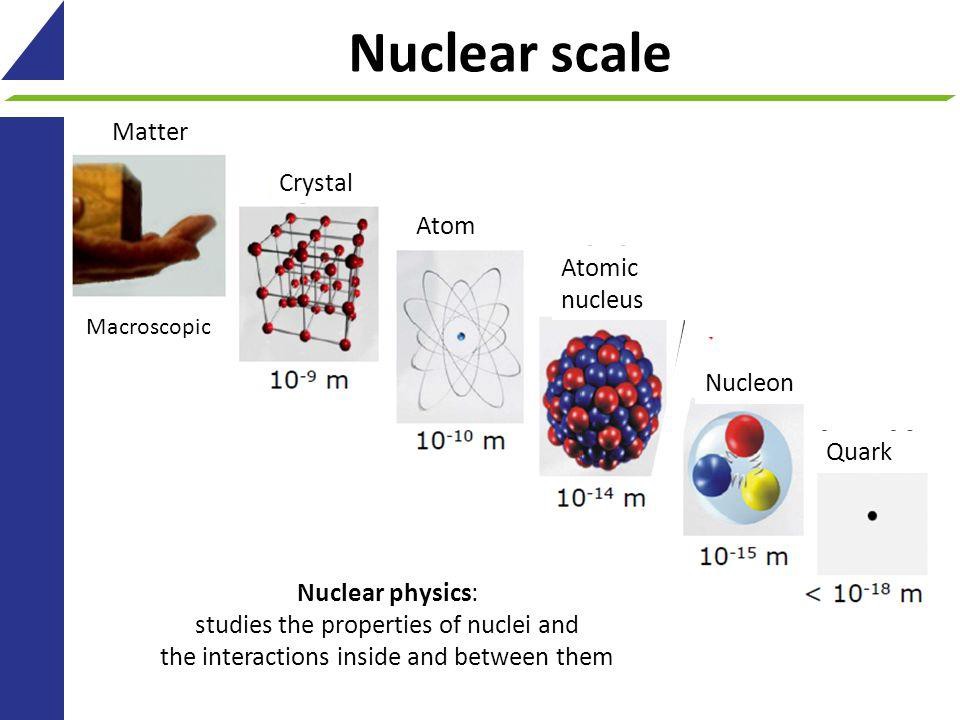
The atoms that are bound together — into molecules and then into larger structures — have restrictions on how their electrons can move. Even when shared between multiple atoms, the electrons orbit in cloud-like shells, and have a smeared-out distribution over time, dependent on what specific energy level (and molecular/atomic orbitals) they occupy. Whether you’re looking at a single atom or a larger structure made out of atoms, that’s the basic picture: there’s a negatively-charged electron cloud orbiting around a single or a series of multiple positively-charged atomic nucleus/nuclei.
So what happens, then, when you bring two atoms close to one another, as you might imagine happens when you bring your thumb and forefinger close to one another, but not so close as to have them touch?
It’s an interesting problem that most physics students learn how to solve in graduate school, where we all get the same answers if we perform our calculations correctly: the shape of the electron cloud that orbits around the atomic nucleus changes in response to the presence of the other nearby atom. Even though atoms (and molecules) themselves are neutral entities, the fact that they’re made of negatively and positively charged components allows them to do something extremely important: to polarize.
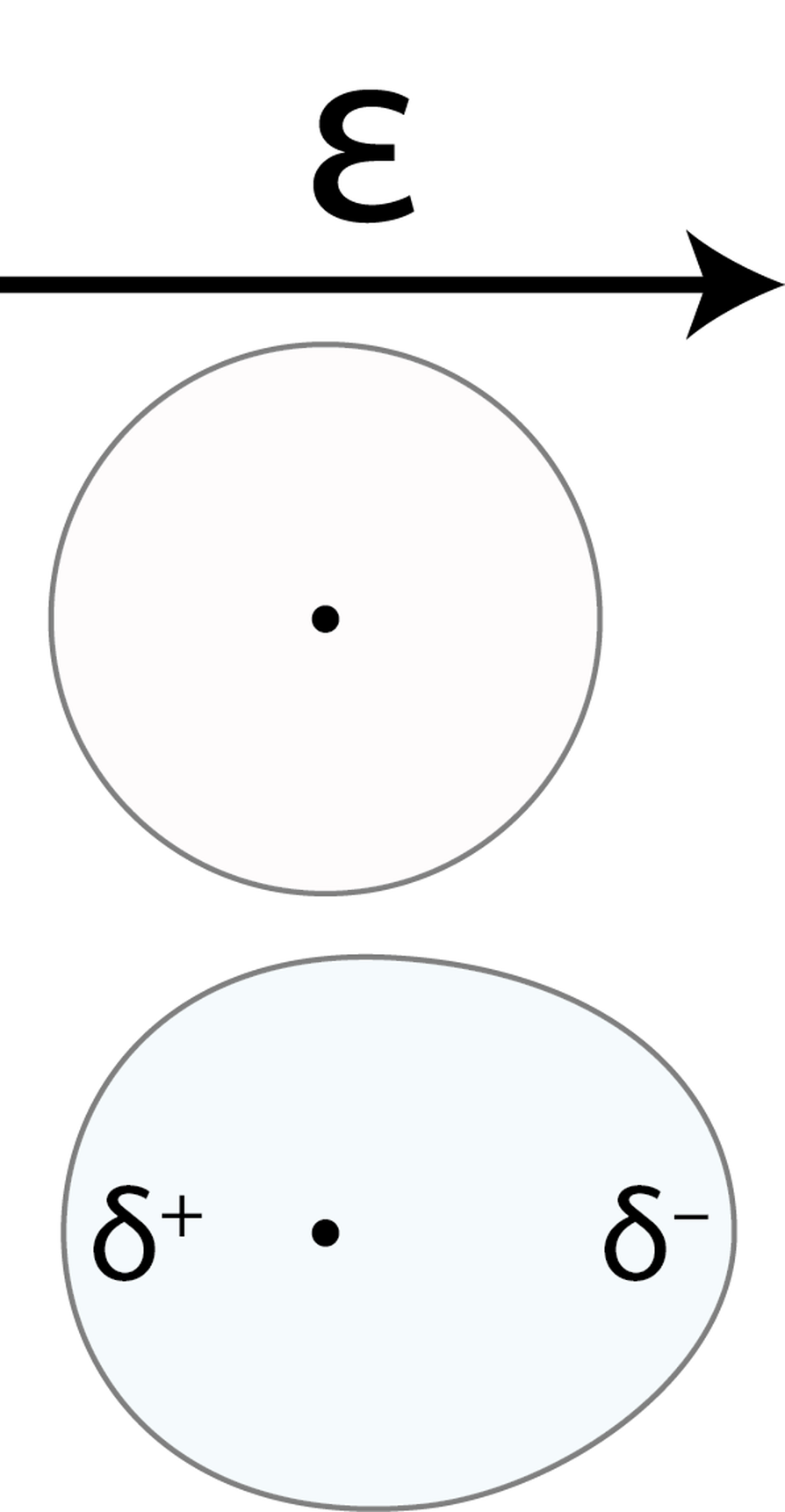
Polarization is a classical electromagnetic phenomenon, occurring wherever you have positive and negative charged together and the ability to have these charges move around and redistribute themselves relative to one another, dependent on the external forces acting on them. It turns out that while having a positive or negative charge nearby is an easy “external force” to visualize, simply bringing two uncharged but polarizable objects close to each other can, in fact, result in not only both objects polarizing, but in a net force being created between the two.
For example, let’s think about two simple atoms that are brought near to one another. Each one has a positively charged atomic nucleus and a diffuse cloud of negative charge around it. If you bring one into the vicinity of the other, they’ll initially remain spherical: with no net attractive or repulsive force. However, the closer you bring them together, the more the electron clouds will distort in their shape, creating a tiny dipole: where one positively charged nucleus is slightly off-center relative to the negatively-charged spherical distribution of the negative charges.
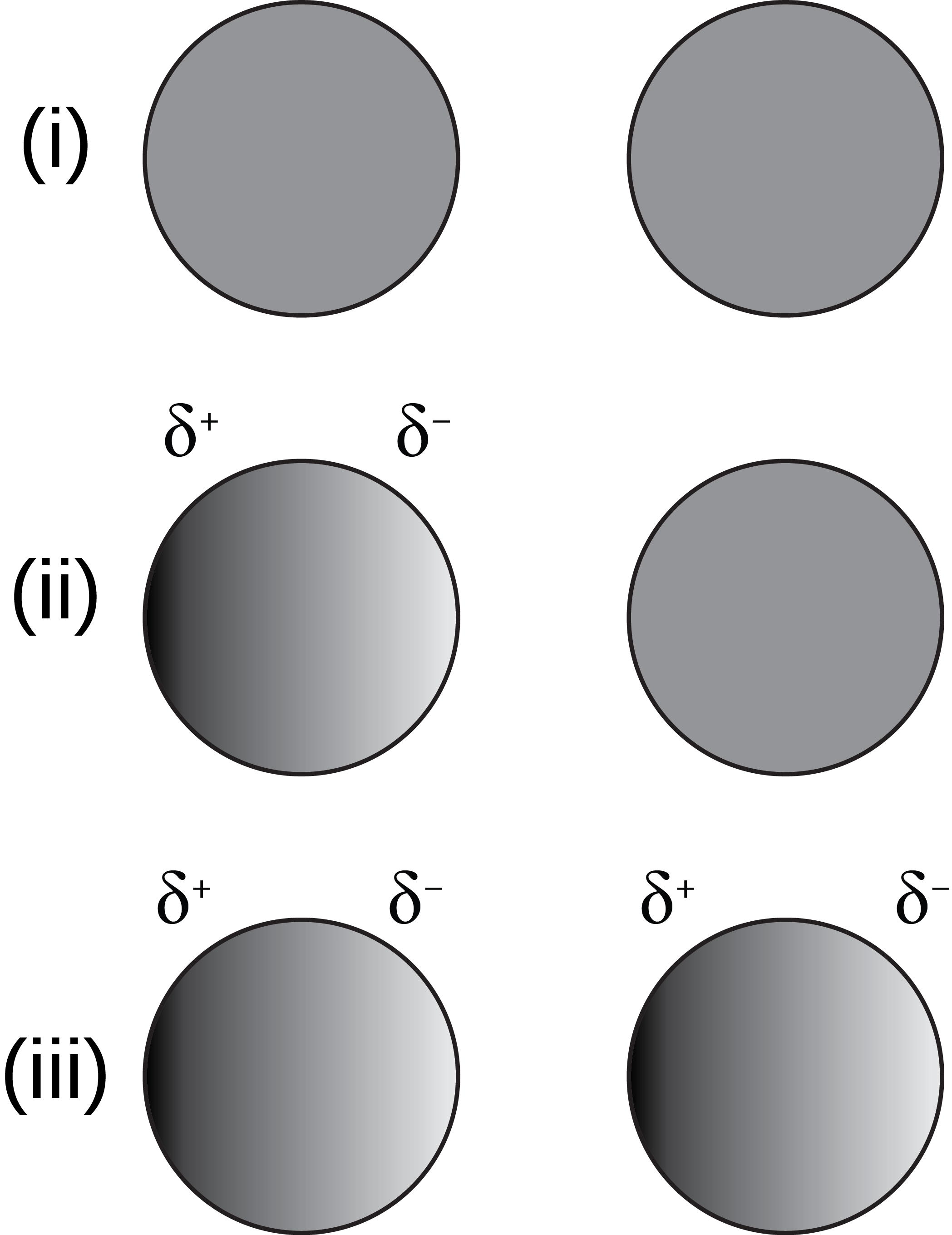
As soon as you have one atom behave as an electric dipole — to be polarized — then it starts generating its own electric field, which polarizes any atoms in its vicinity. If the “positive” end is closer to the other atom, then it pushes the “positive” nucleus farther away and draws the “negative” electron cloud closer to it, leading to an attractive force between the two atoms. This attractive force, which can be experienced at short distances, is known as the Van der Waals force, and explains why, when you rub a blown-up balloon on your shirt (and transfer some electrons to it), you can “stick” the balloon to the wall where you rubbed it: because the charged-up balloon polarized the atoms in the wall.
But that was the story for two free, unbound atoms. What if the atoms are bound together in a network of atoms — i.e., in a molecular or a larger structure — where the electrons aren’t entirely free to move, but have some constraints on where they can/can’t go? When one gets brought near the other, now here’s what happens:
- The negatively-charged electrons, where the electron “clouds” overlap, push away from each other, creating an oval-shaped distribution that bulges on the “away from each other” side.
- The positively charged nuclei, because they’re now relatively “closer” to one another on account of the electron clouds polarizing, push away from one another as well.
- And the closer you force them together, the more you increase this effect, causing the repulsive forces to increase even further.
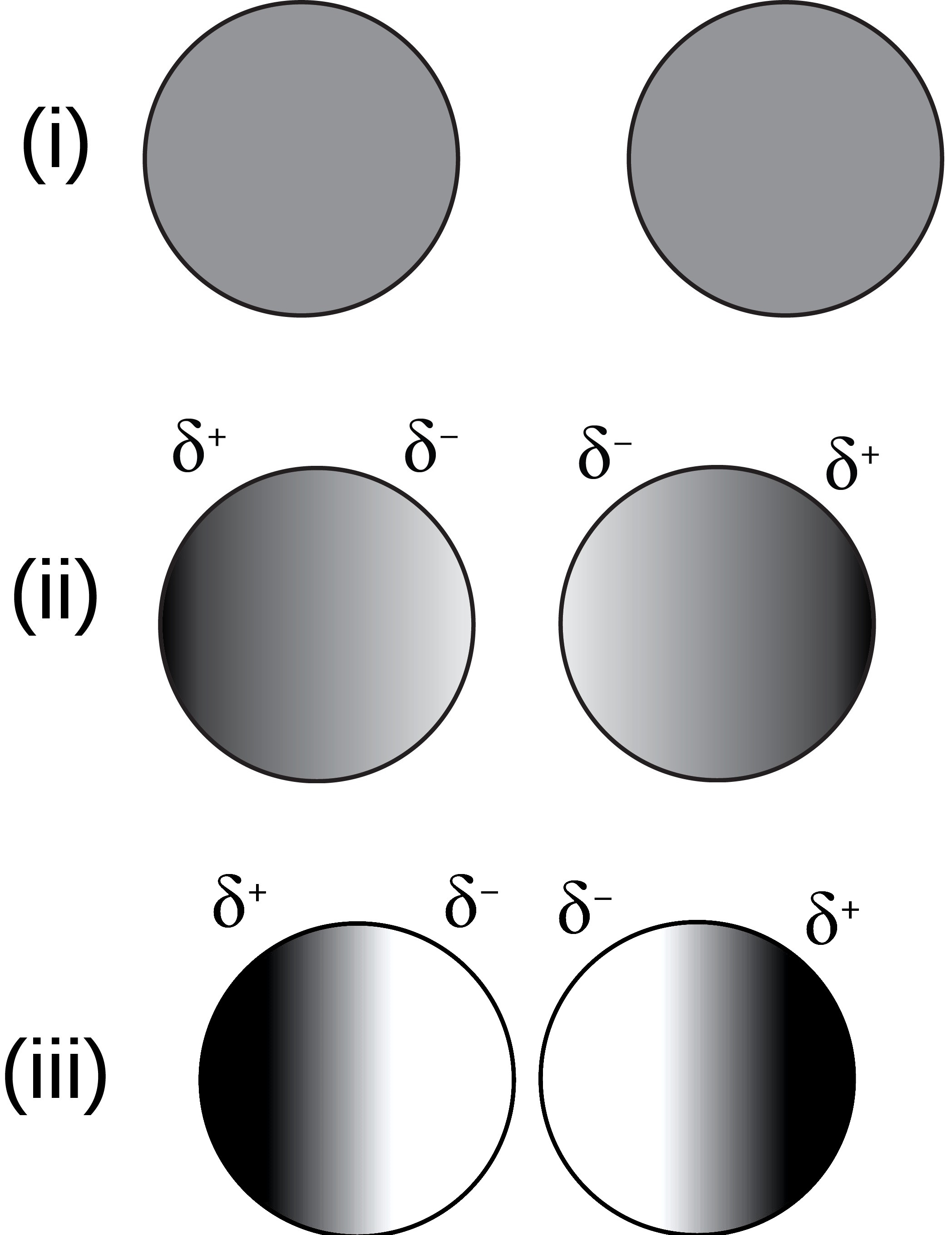
It might seem counterintuitive, but when you bring your thumb and forefinger close together, then have them touch, then push them together with greater and greater amounts of force, this is precisely what’s happening on an atomic/molecular level. However, there’s an extremely important caveat here: this only works, as far as “touching” goes, because the atoms within your thumb are bound to one another much more strongly and securely than they can be “touched” by the atoms in your forefinger. Similarly, the atoms in your forefinger are bound to one another — in molecules, cell membranes, etc. — more strongly than they get “touched” by your thumb.
This is the primary reason why, when you touch two typical objects together, they remain two independent objects, rather than either fusing or merging together. Solid objects, like your finger, have strong atomic bonds — covalent molecular bonds, where the electrons are shared between atoms — that are easy to remain intact and are difficult to destroy. When you push two separate objects together, each object is much more likely to hang onto their own electrons than they are to exchange electrons between them, or to form new covalent bonds from one side to the other.
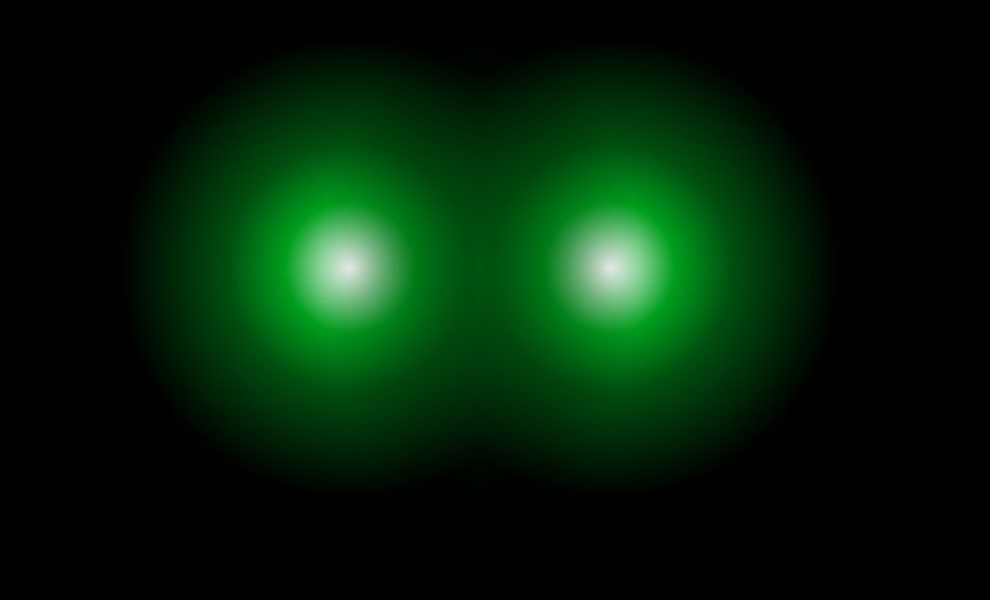
There are exceptions to this, however. If you go outside into cold, sub-freezing temperatures and lick your finger, and then touch your finger to a cold metal surface (do not lick the surface with your tongue!), the water will freeze, with the frozen water bonded to the metal and to the water molecules in your finger. Once you start forming these strong bonds, including:
- ionic bonds,
- covalent bonds,
- or, most strongly, forming a lattice structure that overlaps both objects,
it’s no longer a certainty that individual objects will retain their integrity.
This might seem like an extreme example that couldn’t possibly happen from simply touching your thumb to your forefinger, but if you’ve ever done an extraordinary amount of physical activity with your feet compressed by either being taped up or wedged into a very tight shoe — like a ballet dancer — you might actually be familiar with this phenomenon. Your individual toes, in this case, can wind up binding together in a variety of painful ways, which is why many dancers have begun using toe spacers: to combat the foot deformities that can arise from these mechanical stresses.
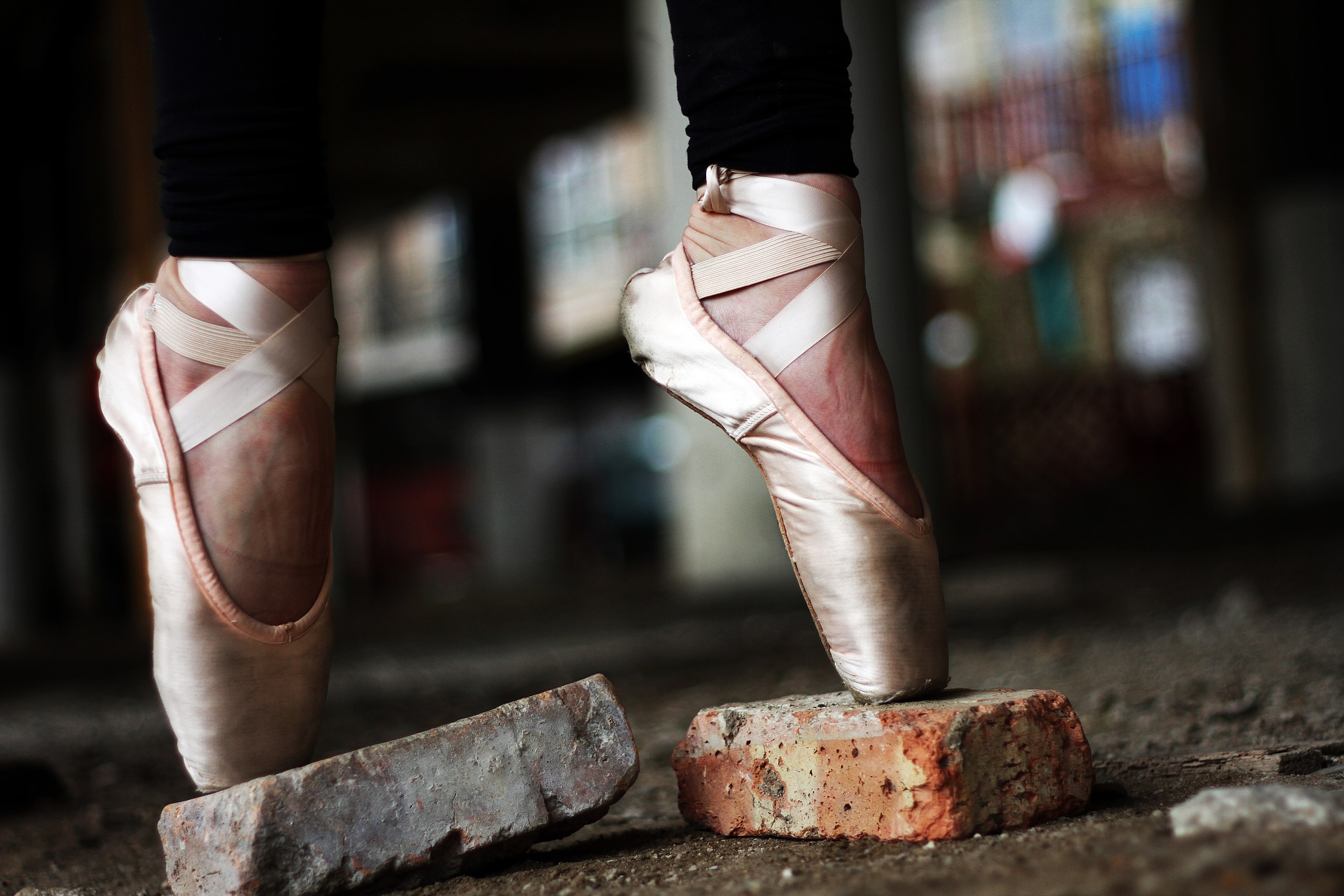
Thankfully, this isn’t something most people have to worry about when they do something mundane like bring their thumb and forefinger together. While you might be able to visually perceive separation distances down to as little as about a tenth of a millimeter (0.0001 meters), there’s a long way down to the size of a typical atom’s electron cloud, which clocks in at an ångström, or one ten-billionth of a meter (0.0000000001 meters).
If you want to know how close you have to bring two atoms so that one starts to polarize, or “respond” in any way to the presence of another, we can estimate that as being about one hundred-millionth of a meter: 0.00000001 meters, or ~10 nanometers: the scale of a fairly large molecule. On this scale, hydrogen bonds can form, meaning that atoms that are polarized in one direction or another within molecules can exert forces that you might very well “feel” with your body.
As you push your fingers together harder and harder, however, the atoms in your thumb and forefinger don’t actually get much closer than that.
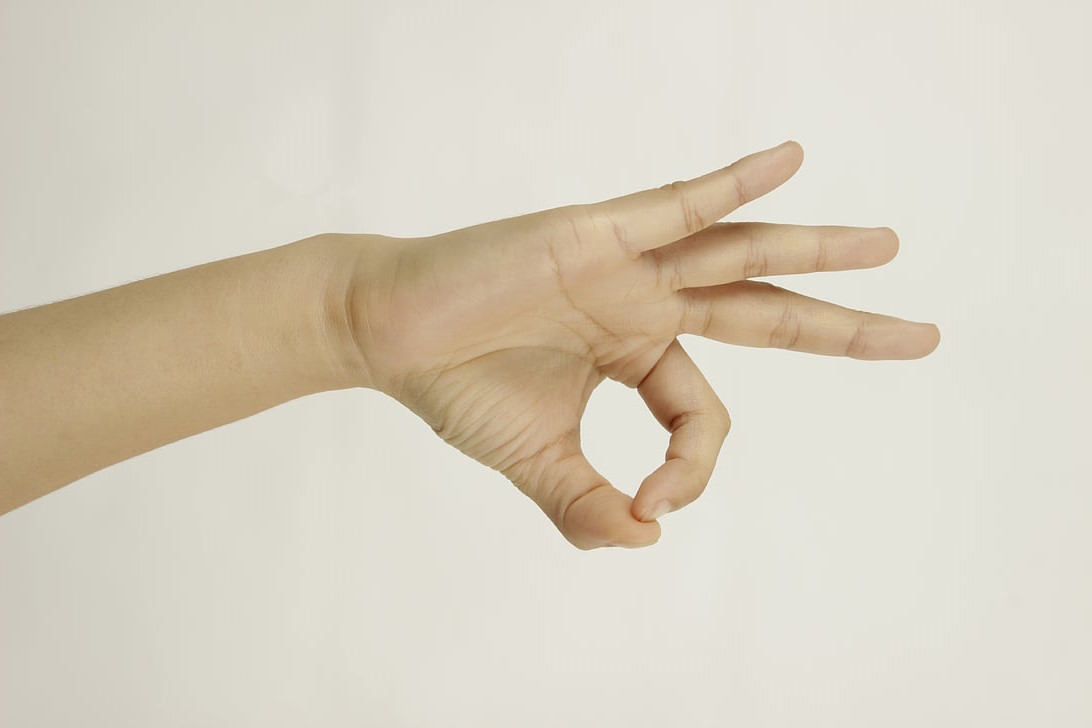
Instead, the bound structures within each one of your fingers — your molecules, the cells that they compose, and the entire cellular structure that makes up each finger — are very strongly (covalently) bonded together. When you push your thumb and your forefinger together, what you’re doing is bringing more and more of these surface atoms into close proximity to one another, and those atoms, being connected to everything else inside your thumb and forefinger, respectively, press against each other.
Even though you can press and exert quite a substantial force on your thumb and forefinger from pressing them against one another — enough to cause your skin to change color, visibly — that force is distributed over a significant area: the area over which your thumb and forefinger touch one another. Forces acting over an area make a pressure, and even though the force is very large, because the area is also large, the pressure is relatively small. As a result, the individual atoms making up your thumb and the atoms making up your forefinger never get extremely close compared to the bond length between atoms within your thumb and forefinger individually.
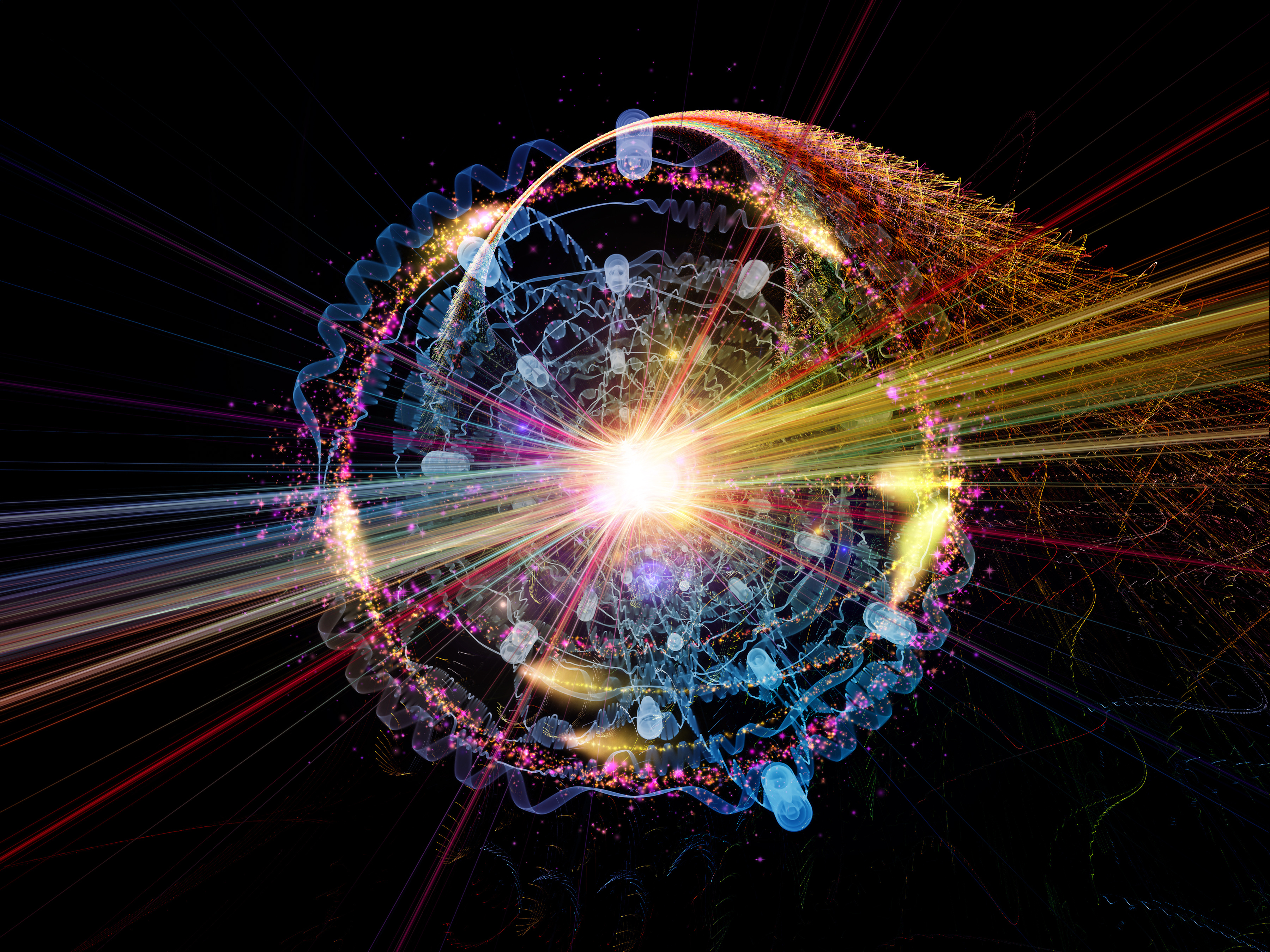
This also answers a question that many people often wonder: if my atoms are mostly empty space, why won’t my thumb and forefinger ever pass through one another when I bring them together? Although many people rush to a quantum rule — the Pauli Exclusion Principle — that’s not actually necessary. The integrity of atoms, the fact that they’re covalently (strongly) bonded to one another in molecules, and the fact that negative electron charges are distributed over a large volume of space is more than sufficient to prevent two atom-based structures from passing through one another. Chemical, electron-based bonds, and the large spatial distribution that the electrons occupy, is enough to cause matter to take up space.
But that’s the key: when we say “touch” one another, we really just mean “How close does something need to get so that its properties become something my sense-of-touch, or the nerves within my body that are sensitive to that sensation, respond to it?” And while we have different neurons that are sensitive to temperature, pressure, and pain, they’re all triggered by either electrons or photons interacting with the matter in our bodies. In the case of pressure-based touch, a distance that’s significantly less than your eye can see, but still substantially greater than the size of an atom, is all that’s required to instigate a response!
Send in your Ask Ethan questions to startswithabang at gmail dot com!