Why The Universe Probably Isn’t Shaped Like A Donut
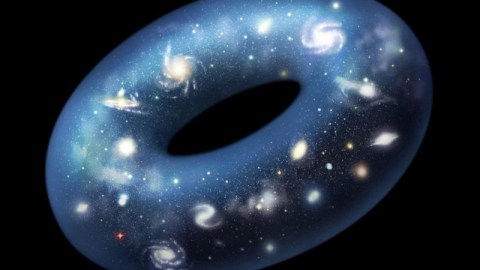
Despite the recent headlines, it’s an extraordinarily unlikely proposition.
Have you ever wondered, if it were possible to travel through space straight ahead as quickly as you could imagine, if you wouldn’t someday return to your original starting point? Here on Earth, if you could walk in a straight line for some 40,000 kilometers, ignoring obstacles such as oceans and mountains, you’d return to your starting point. The Earth, indistinguishable from “flat” on the scale of your own backyard, is both finite in extent and also simply connected, meaning that any loop you draw on it can be contracted down to a single point.
The Universe, on the scales that we can observe it, also appears indistinguishable from flat: we detect no trace of spatial curvature even on the largest cosmic scales we can access. It’s possible that the actual Universe, beyond the limits of what we can observe, remains flat and extends arbitrarily far — perhaps even infinitely — in all directions. But it’s also possible that out there, beyond the limits of what we see, the Universe is finite, either curved on some large scale and simply connected or even flat, but part of a multiply connected, donut-like space.
It’s a fascinating idea, one that’s just had new life breathed into it. But is it really backed up by the scientific evidence? Here’s what we know today.
The Universe, as we see it today, presents us with a number of clues about its past history. The galaxies we see in the night sky are full of stars all their own, located millions or even billions of light-years away; the Milky Way is one of perhaps 2 trillion galaxies we’re capable of observing. The farther away these galaxies are from us, the greater the amount that their light is shifted towards longer, redder wavelengths. This teaches us — combined with Einstein’s General Relativity — that the Universe is expanding today, and therefore should have been denser, hotter, and more uniform in the past.
Extrapolating backwards, you can imagine a time where things were so dense and so hot that anytime an electron and an atomic nucleus found one another, they’d attempt to form a neutral atom, but that success would be very short-lived. Almost immediately, another particle or photon would come along with enough energy to kick the electron off of that atom, ionizing it once again. It’s only when the Universe cools sufficiently that the remaining photons don’t have enough energy to ionize those atoms that we get our first light signal from the Universe: the Big Bang’s leftover glow, visible today as the cosmic microwave background (CMB).
When we see this glow, we see it omnidirectionally: it comes no matter where we look in space. Even though the temperature is low today, at just 2.725 K, it’s incredibly uniform, with “hot spots” and “cold spots” differing from the average temperature by merely ~100 microkelvin or so: about 1-part-in-30,000. And we can also examine the details of differently-sized regions, to determine if there are scales above which temperature fluctuations suddenly cease to exist.
Why would there be such a scale?
Well, for one, because the speed of light is finite. If the Universe began in an instant at the moment of the Big Bang, even if it’s been expanding ever since, there ought to be a limiting scale — particularly in the Universe’s past — where no signals, even traveling at the cosmic speed limit, could have reached from one region to another. We’d expect that there might be a cutoff to how large a scale we see these temperature fluctuations on: the scale of the cosmic horizon. Above such a scale, the Universe shouldn’t have these coherent fluctuations; you’d expect there would be no such thing as super-horizon fluctuations.
Of course, super-horizon fluctuations do exist, as confirmed by the polarization data of the CMB: first by WMAP and later (and to greater precision) by Planck. This is one of the great pieces of observational evidence supporting cosmic inflation and disfavoring the idea that the Big Bang represents a singular origin for our Universe.
Another thing that’s encoded in the CMB — in the temperature fluctuations, rather than the polarization data — is how the magnitude of the fluctuations, or the differences between the hot/cold spots and the average temperature, changes as a function of angular size.
You can imagine putting down a circle of a particular size over a map of the CMB, and taking the average temperature inside that circle. On smaller angular scales, you have many, many regions to sample; on large angular scales, you only have a few. The geometry of the Universe determines whether these fluctuations appear to be:
- their actual size,
- smaller than their actual size,
- or larger than their actual size,
dependent on the curvature of space. To the best of our precise measurements, which means to a precision of better than 1-part-in-250, the entire observable Universe is indistinguishable from spatially flat.
This leaves us with a few possibilities for what’s actually going on with the Universe. They are as follows:
- The Universe is perfectly spatially flat, and never loops back on itself or reconnects; it’s flat and infinite in extent.
- The Universe is actually curved — either positively like a (higher dimensional) sphere or negatively like a horse’s saddle — but the scale of its curvature is so large, at least hundreds of times the scale observable to us, that it appears indistinguishable from flat.
- Or the Universe is perfectly spatially flat, but it has a non-trivial, multiply-connected topology. It’s finite in extent, but appears flat everywhere we look.
That last possibility is an exotic one, but one worth considering because it could potentially lead to observable effects. One test would be to probe the fluctuation patterns in the CMB to look for signs that could identify temperature patterns in one location with the same patterns elsewhere. If the Universe looped back on itself, where traveling far enough in one direction would lead you back to your starting point, these repeating patterns would appear in the CMB if the size of the Universe were smaller than the scale of the cosmic horizon.
We’ve searched for those features, and they don’t exist. If the Universe does loop back on itself, it happens on cosmic scales that are larger than the ones we can observe. But that isn’t the end of the line for this option, because there can be a relationship between the geometry of the Universe and the scales — scales above the early cosmic horizon — on which temperature fluctuations occur.
According to inflation, the Universe should have been “seeded” with temperature fluctuations on all cosmic scales, and the magnitude of those fluctuations should be almost perfectly the same across all cosmic scales. Smaller scales will have time to experience the effects of gravitation, of radiation pressure, and of collisions between photons and normal matter, while larger scales will not. This means we expect to see, on small scales, a series of peaks and valleys, but on large scales, the spectrum of temperature fluctuations should be constant.
However, there’s a small discrepancy between what we naively expect the Universe to be like compared to what we actually see, and that’s what we need to pay attention to.
On the very, very largest of cosmic scales, on angular scales of 60° or greater, we find that the temperature fluctuations — the amount that the actual temperatures in the Universe deviate from that 2.725 K average — are actually lower than we expect. Instead of deviating from the average by ~100 microkelvin or so, they only deviate by somewhere around ~20-to-30 microkelvin, a very small value. It’s so small that, for some time now, it’s led astronomers and astrophysicists to question whether there’s a physical reason behind it.
There may not be one, of course. The predictions that we make for what we ought to observe are only statistical predictions: if we had an infinite number of Universes created by the processes that we think created ours, we know what we’d expect to observe. However, we only have one Universe to observe, and on the largest cosmic scales, where we have the smallest number of independent regions, we simply get what we get. The odds of winding up with a Universe where the largest angular scales have temperature fluctuations as minuscule as ours are low, but not absurdly so: about 1-in-800, or a little better than 0.1%.
With such low statistics to sample from, it’s practically impossible to draw any definitive conclusions for why the Universe has these particular properties. Still, it’s worth considering whether there might be a physical mechanism that causes these large angular scales to have such small temperature fluctuations. In 2003, a research team led by Jean-Pierre Luminet discovered a brilliant possibility: that if the Universe, rather than being smooth, instead had the (topologically) mathematical shape of a dodecahedron — a 12-sided regular polyhedron — it could suppress the temperature fluctuations that appeared on the largest cosmic scales.
While certain other predictions of that model didn’t quite pan out, it brought a previously obscure line of thought into the mainstream: that if the Universe isn’t simply connected, where any circle you drew could be shrunk down into a point, but multiply connected, where some circles would be unable to be shrunken beyond a certain length, that could suppress the temperature fluctuations on the largest of cosmic scales.
And what’s the simplest example of a flat, multiply-connected, three-dimensional space? A torus, whose shape most commonly resembles a donut: the kind with a hole in the center.
That’s precisely what the latest study is about that’s sparking the recent headlines: the revival of an 18-year-old idea in a slightly different incarnation. Much like the idea that the Universe could have the topology of a dodecahedron, the idea that the Universe has the topology of a donut does come along with implications for what we should observe, but these too are only implications in a statistical sense. Dependent on the size of the donut/torus, particularly if it’s only a little bit larger than the observable part of our Universe, its predictions are slightly more consistent with our observations than a flat, simply-connected Universe that requires this ~0.1% likelihood to have been spontaneously realized.
Because it accounts for the suppressed power on these large angular scales, the idea is definitely worth keeping an eye on. However, this violates the cardinal rule of a compelling new theoretical idea: you must not invoke one new parameter to better account for one unanticipated observation. In theoretical physics, we demand predictive power. If you’re going to add a new ingredient to your Universe, it had better:
- reproduce all the successes of the old theory,
- account for the observations the old theory could not,
- and make new, testable predictions that differ from the predictions of the old theory.
Add-ons that fold in one new parameter to account for one new observable are a dime-a-dozen, unfortunately, and that’s all this “new proposal” does.
The true problem with the Universe is that there’s only one to observe, or at least, only one that we’re capable of observing. We don’t have a large sample of Universes to compare between, and we don’t have a large set of data points available to us within our Universe. It’s like rolling five dice, together, once. Your odds of getting all sixes is small: about 1-in-7800. Yet if you rolled five dice at once and saw that it came up all sixes, you wouldn’t necessarily conclude that it was anything more than random chance. Sometimes, nature just doesn’t give you the most likely outcome.
It’s possible that the leftover photons from the Big Bang, reaching us today as a snapshot from 13.8 billion years ago, really are the result of expanding from a donut-shaped Universe, one that’s barely larger than the observational limits of what we perceive today. But the one piece of evidence we have to support that scenario isn’t particularly compelling, and cannot rule out the null hypothesis: that we live in a Universe indistinguishable from flat, simply connected, and without any fancy topological traits. Unless we find a way to extract more information from our Universe — and we’ve already pulled everything out of the cosmic microwave background that we can, to the limits of our observations — we may never be able to meaningfully discriminate between these two possibilities.
Starts With A Bang is written by Ethan Siegel, Ph.D., author of Beyond The Galaxy, and Treknology: The Science of Star Trek from Tricorders to Warp Drive.