Why Is It So Hard To Find A New Particle?
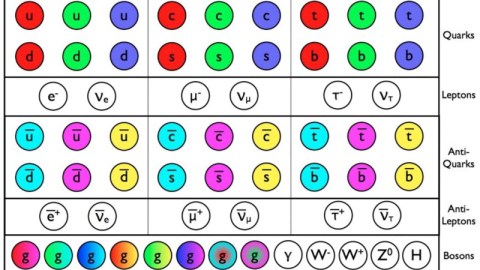
We know the Standard Model isn’t all there is. So why haven’t we found a single particle outside of it?
“I often feel a discomfort, a kind of embarrassment, when I explain elementary-particle physics to laypeople. It all seems so arbitrary — the ridiculous collection of fundamental particles, the lack of pattern to their masses.” –Leonard Susskind
When we take a look at some of the greatest unsolved problems in theoretical physics today, there’s something many of them have in common.
- Why is the Universe full of matter, but not antimatter?
- What is the nature of dark matter?
- What mechanism gives neutrinos their uniquely small (but non-zero) mass?
- And why do the weak nuclear interactions violate a special symmetry, but not the strong interactions?
If the Standard Model were all there was in the Universe, we wouldn’t be asking all of these questions.
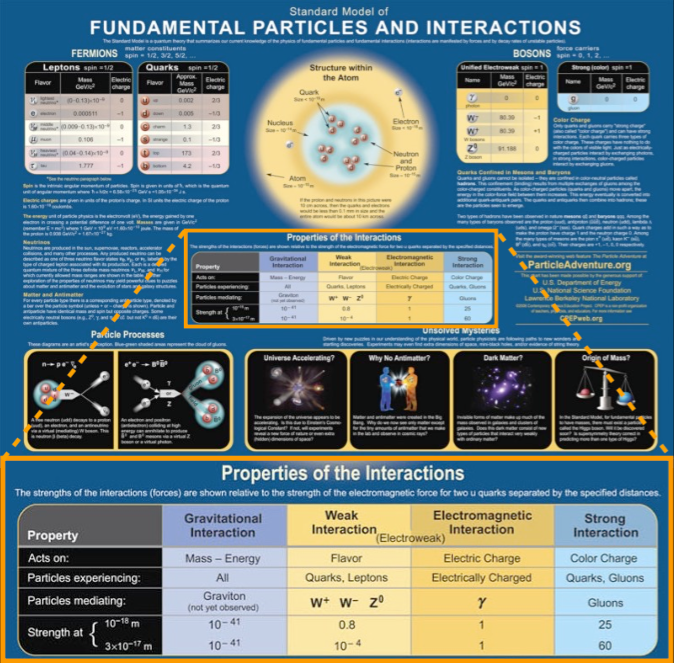
According to the known particles and interactions, there should be an equal amount of matter and antimatter, yet our Universe has a fundamental asymmetry here. If all we had were Standard Model particles, we wouldn’t see galaxies, clusters and the large-scale structure of the Universe behaving as it does; that requires dark matter. Neutrinos should be completely massless, and yet the observed phenomenon of neutrino oscillation shows us they not only have mass, but that mass is millions of times smaller than the next lightest known massive particle. And CP-violation is explicitly allowed in both the weak and strong nuclear interactions, but our Universe only seems to exhibit it in weak decays.
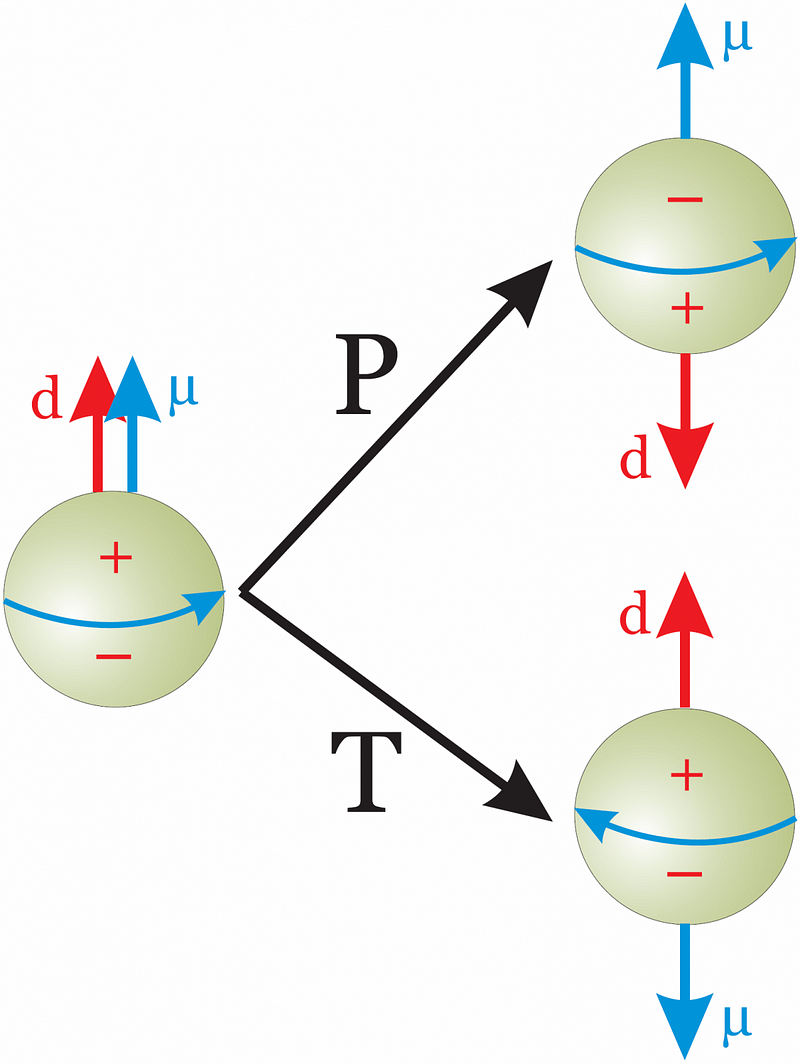
These four problems all have something in common: they can all be solved by adding new, beyond-the-Standard-Model particles. In fact, for most of these problems, any (workable) theoretical solution we’ve been able to contrive mandates the existence of new particles. And new particles — if they exist — are surprisingly easy to make.
All you have to do is take matter and antimatter, collide them together at high energies, and so long as you have more energy than you need to make such a new particle, where that energy is given by E = mc2, then sometimes at those high energies, it will simply pop into existence! Yet, in the last 50 years, colliders have gotten more and more powerful, from just a few MeV (mega, or one million, electron-Volts) into the GeV range (giga-electron-Volts, or billions of eV) and, with the advent of Fermilab and now the Large Hadron Collider, we’ve crossed over into the TeV (tera-electron-Volt, or trillions of eV) range.
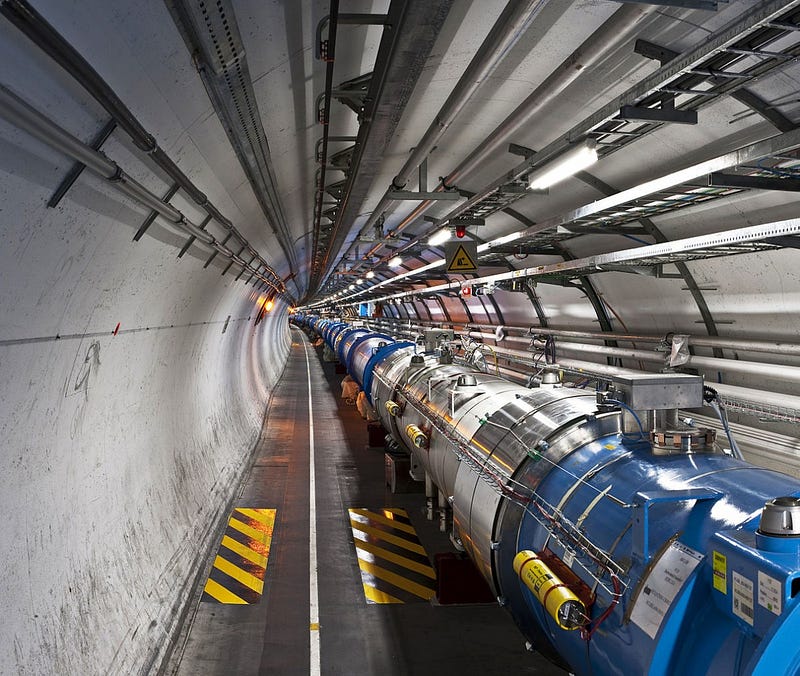
Although colliding particles at these energies — and building tremendous, sophisticated detectors around the collision points — has enabled us to find every single particle and antiparticle predicted by the Standard Model, we’ve thus far found nothing beyond it. As a result, theorists have cooked up a whole slew of scenarios that could still solve problems such as these, but that make the particles more difficult to find. Most commonly, we create models where either we simply haven’t obtained the proper energies to find these particles, or where the particles are hidden or decoupled from the standard three (electromagnetic, weak nuclear and strong nuclear) forces.
Some common options include:
- supersymmetry, where the lightest supersymmetric particle is still out of range from what the LHC should find,
- sterile neutrinos, where there are additional neutrinos that interact with other neutrinos, but don’t interact with other matter via the three main forces,
- grand unification, where super-heavy particles couple to the Standard Model particles, but don’t exist at our lower-energy scales,
- extra dimensional particles (Kaluza-Klein particles), where higher energies will reveal these beyond-the-current-LHC-limit particles,
- or technicolor/leptoquark-inspired theories, where additional fundamental particles exist at high energies, either in addition to or inside of Standard Model particles.
But there’s an extra problem that limits most examples of all of these models: known physics is measured extremely well, and two things in particular require that the Universe doesn’t deviate from the Standard Model all that much.
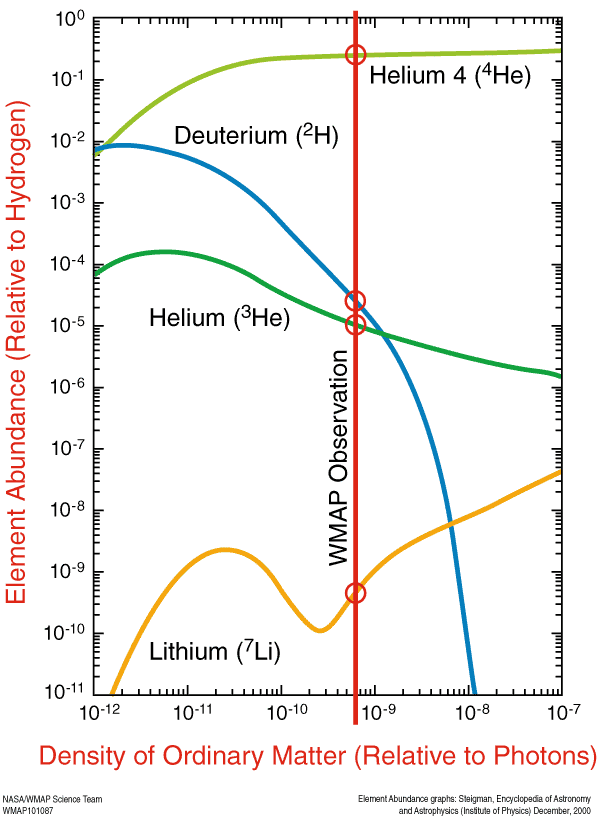
1.) Big Bang Nucleosynthesis works really, really well. In the Early Universe, back during the first few minutes after the Big Bang, energies were incredibly high, temperatures were really hot, and particles were just being formed. There’s a period where we formed neutrons and protons for the first time, in roughly a 50/50 split. When things were very hot, protons could combine with electrons to form neutrons and neutrinos, just as neutrons and neutrinos could combine to form protons and electrons.
But as the Universe cooled, it became easier for neutrons and neutrinos (because they’re heavier) to form protons and electrons than the other way around, turning that 50/50 split into an 85/15 split, in favor of protons. Some 3–4 minutes later, nuclear reactions could finally proceed, but not until about 20% of those neutrons decayed, giving us an 88/12 split. The observed helium-to-hydrogen ratio left over from the Early Universe agrees with Big Bang nucleosynthesis tremendously well, putting strict limits on any beyond-the-Standard-Model particles that would alter that reaction.
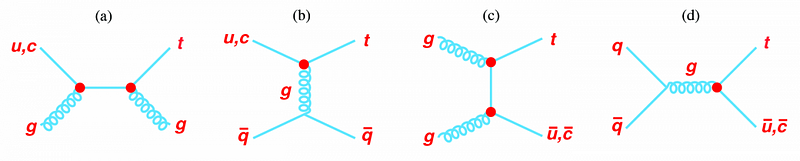
2.) There’s no such thing as a flavor-changing-neutral-current (FCNC). There are six types of quarks and six types of leptons, and they come in three generations:
- Generation 1, containing the up and down quarks, the electron and the electron neutrino.
- Generation 2, containing the charm and strange quarks, the muon and the muon neutrino.
- Generation 3, containing the top and bottom quarks, the tau and the tau neutrino.
While any particle in generation 3 can decay to a generation 2 or 1 particle, and any particle in generation 2 can decay to a generation 1 particle, we’ve only ever seen these decays mediated by a charged particle (like a W-boson), never by a neutral particle (like a Z-boson). The limits from colliders on these decays are extraordinarily strict, and so the lack of FCNCs seems to be a simple fact of nature.
Yet almost all of the extensions to the Standard Model we consider — including most models of supersymmetry, extra dimensions, and grand unified theories — contain FCNCs, and contain far too many of them to be consistent with the Universe we observe.
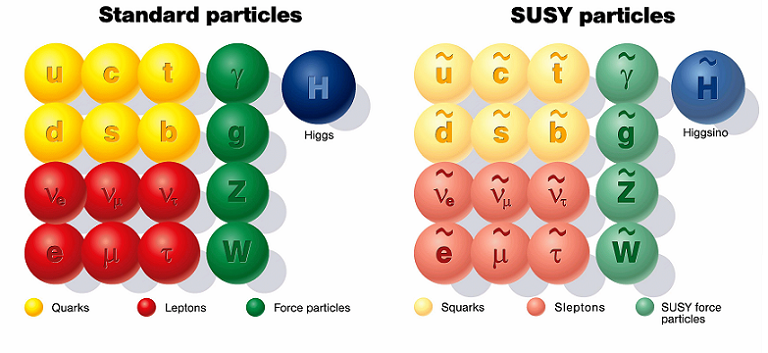
The success of the Standard Model is both a blessing and a curse. It’s a blessing that we’ve uncovered a theory that describes nature so well, and that appears to work for all the particle decays and interactions we’ve ever seen so far. But it’s a curse, in that we know there must be more Universe out there, as there are questions the Standard Model can’t answer. Yet the successes make our options to account for its shortcomings all the more unsatisfying, with a compelling answer yet to come to light.
The search continues, and the best we can hope for is that nature surprises us with an unexpected discovery that points the way forward.
Leave your comments on our forum, help Starts With A Bang! deliver more rewards on Patreon, and order our first book, Beyond The Galaxy, out now!