What Was It Like When Planet Earth Took Shape?
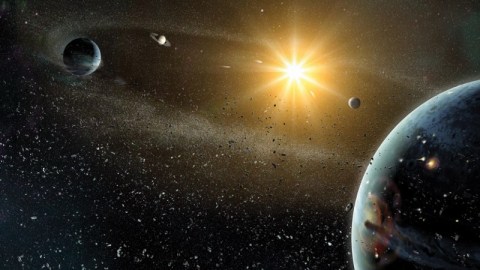
The ‘giant impact’ that led to Earth might not have been so giant, after all.
A little over 4.5 billion years ago, our Solar System began to form. Somewhere in the Milky Way, a large cloud of gas collapsed, giving rise to thousands of new stars and star systems, each one unique from all the others. Some stars were much more massive than our Sun; most were much smaller. Some came with multiple stars in their systems; about half the stars formed all by their lonesome, like ours did.
But around practically all of them, a large amount of matter coalesced into a disk. Known as protoplanetary disks, these would be the starting points for all the planets that formed around these stars. With the advances in telescope technology that’s accompanied the past few decades, we’ve started to image these disks and their details firsthand. For the first time, we’re learning how planetary systems like our own came into existence.
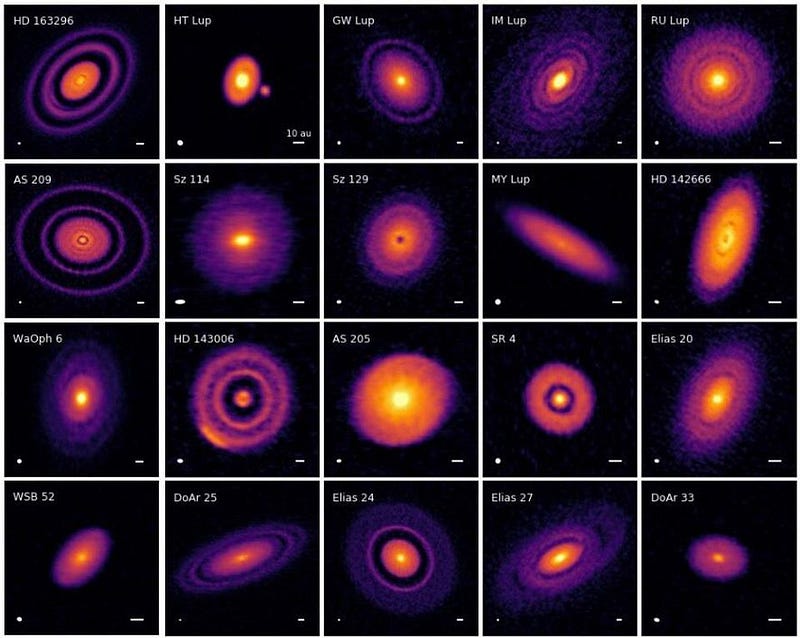
In theory, the process of forming planets is incredibly straightforward. Whenever you have a large mass, like a gas cloud, you can expect the following steps to happen:
- the mass gets drawn into a central region,
- where one or more large clumps will grow,
- while the surrounding gas collapses,
- with one dimension collapsing first (creating a disk),
- and then imperfections in the disk grow,
- preferentially attracting matter and forming the seeds of planets.
We can now look directly at these protoplanetary disks, and find evidence that these planetary seeds are present from a very early time.
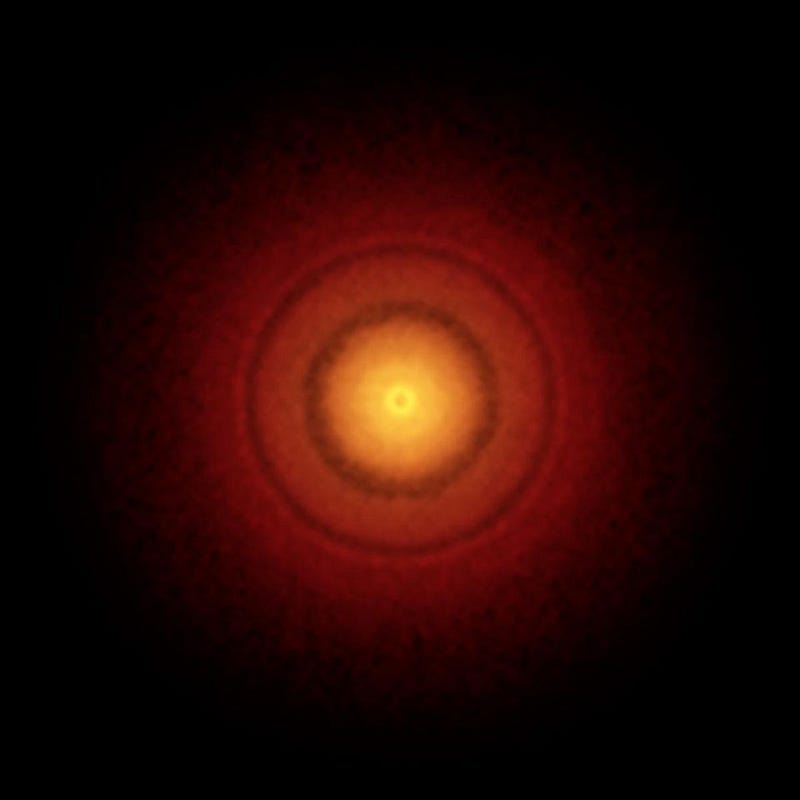
But these disks won’t last very long. We’re looking at timescales that are typically only tens of millions of years long to form planets, and that’s due to not only gravitation, but to the fact that we’ve got at least one central star shining as well.
The cloud of gas that will form our planets is made out of a mix of elements: hydrogen, helium, and all the heavier ones, going way up the periodic table. When you’re close to the star, the lightest elements are easy to blow off and evaporate. In short order, a young solar system will develop three different regions:
- a central region, where only metals and minerals can condense into planets,
- an intermediate region, where rocky and giant worlds with carbon compounds can form,
- and an outer region, where volatile molecules such as water, ammonia, and methane can persist.
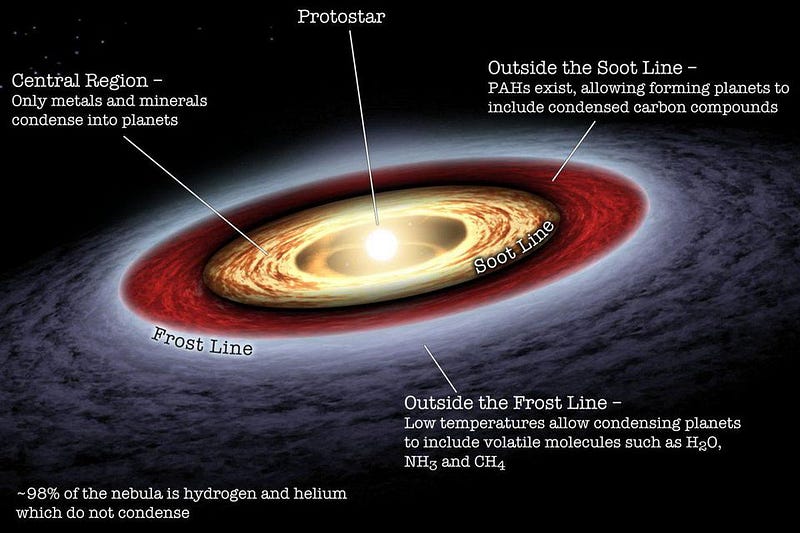
The border between the inner two regions is known as the Soot Line, where being interior to it will destroy the complex carbon compounds known as polycyclic aromatic hydrocarbons. Similarly, the border between the outer two regions is known as the Frost Line, where being interior to it will prevent you from forming stable, solid ices. Both lines are driven by the heat of the star, and will migrate outward over time.
Meanwhile, these protoplanetary clumps will grow, accrete additional matter, and will have opportunities to gravitationally perturb one another. Over time, they can merge together, gravitationally interact, eject each other, or even hurl one another into the Sun. When we run simulations that allow planets to grow and evolve, we discover an extraordinarily chaotic history that’s unique for each and every solar system.
When it comes to our own Solar System, the cosmic story that unfolded was not only spectacular, it was in many ways unexpected. In the internal region, it’s very likely that we had a relatively large world present early on, which was possibly swallowed by our Sun in our cosmic youth. There is nothing preventing a giant world from forming in the inner Solar System; the fact that we have only the rocky worlds close to our Sun tells us that something else was likely present early on.
The largest planets probably formed from seeds early on, and there may have been more than four of them. In order to get the present configuration of gas giants, the simulations we run seem to show that there was at least a fifth giant planet that was ejected at some point long ago.
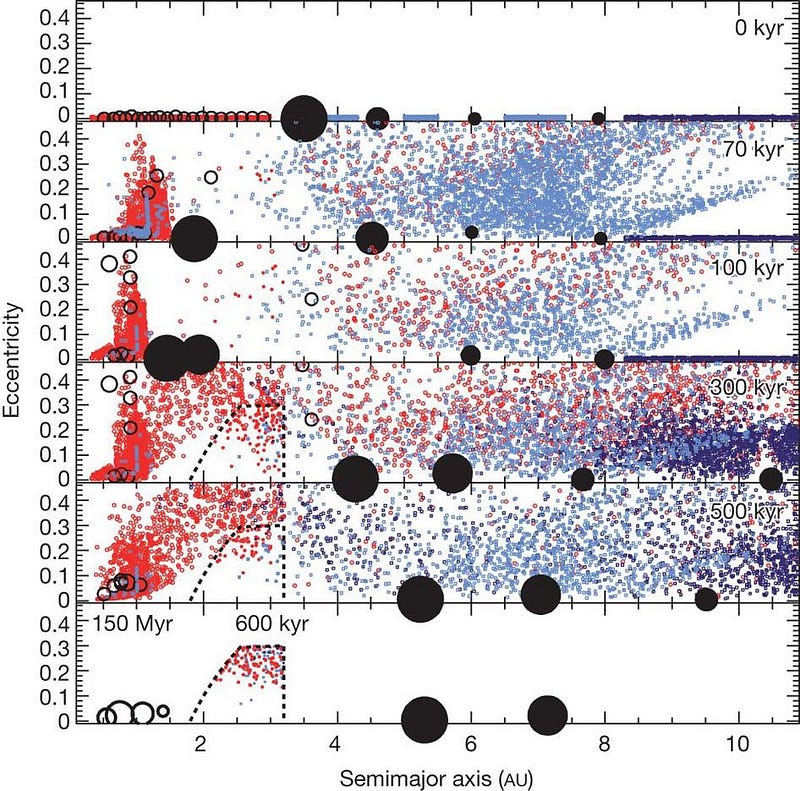
The asteroid belt, between Mars and Jupiter, is very likely the remnants of our initial Frost Line. The border between where you can have stable ices should have led to a large number of bodies that were a mix of ice and rock, where the ices mostly sublimated away over the billions of years that have passed.
Meanwhile, out beyond our last gas giant, the leftover planetesimals from the Solar System’s earliest stages persist. Although they may merge together, collide, interact, and occasionally get hurled into the inner Solar System from gravitational slingshots, they largely remain out beyond Neptune, as a relic from the youngest stages of our Solar System. In many ways, these are the pristine remnants from the birth of our cosmic backyard.
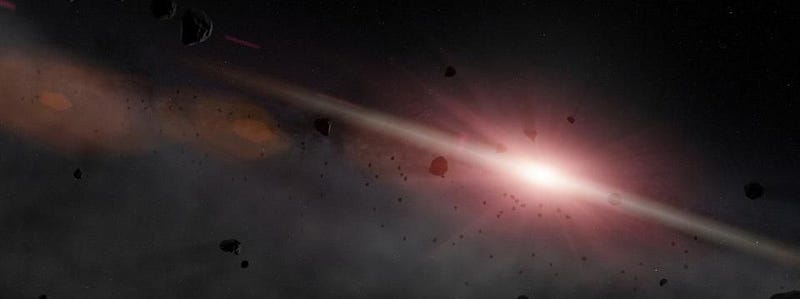
But the most interesting place of all, for our purposes, is the inner Solar System. There may have once been a large, interior planet that was swallowed, or perhaps the gas giants once occupied the inner regions and migrated outwards. Either way, something delayed the formation of planets in the inner Solar System, allowing for the four worlds that did form — Mercury, Venus, Earth, and Mars — to be much smaller than all the others.
From whatever elements were left, and we know they were mostly heavy ones from the planetary density measurements we have today, these rocky worlds formed. Each one has a core made of heavy metals, accompanied by a less-dense mantle made out of material that fell onto the core later, from beyond the Frost Line. After only a few million years of this type of evolution and formation, the planets were similar in size and orbit to how they are today.
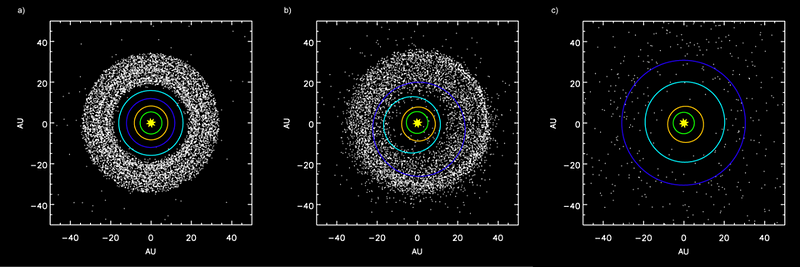
But there was a huge difference: in these early stages, Earth didn’t have our Moon. In fact, Mars didn’t have any of its moons, either. In order for this to occur, something needed to create them. That would require a giant impact of some type, where a large mass struck one of these early worlds, kicking up debris that eventually coalesced into one or more moons.
For Earth, this was an idea that wasn’t taken particularly seriously until we went to the Moon and investigated the rocks we found on the lunar surface. Quite surprisingly, the Moon has the same stable isotope ratios that the Earth does, while they’re different between all the other planets of the Solar System. Additionally, the Earth’s spin and the Moon’s orbit around Earth have similar orientations, and the Moon has an iron core, all facts which point to a mutual common origin for the Earth and the Moon.
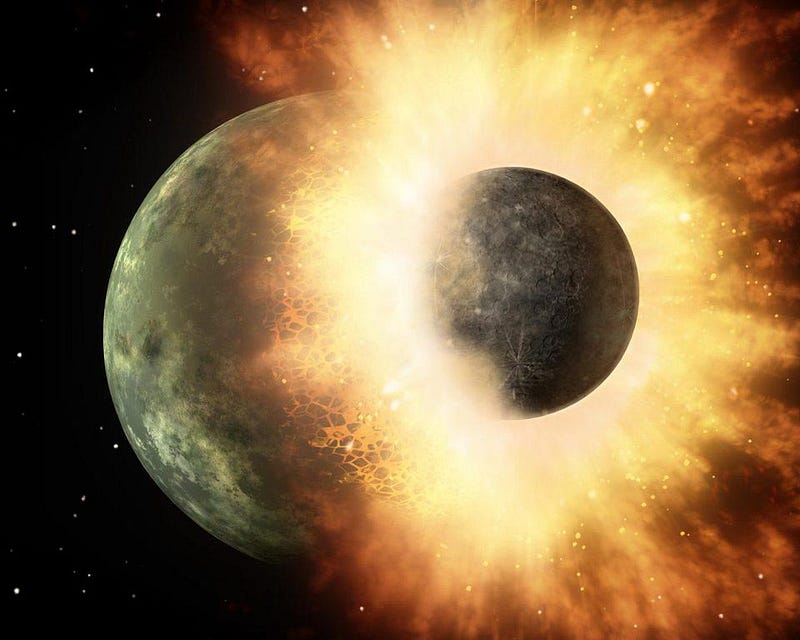
Originally, the theory was called the Giant Impact Hypothesis, and was theorized to have involved an early collision between proto-Earth and a Mars-sized world, called Theia. The Plutonian system, with its five moons, and the Martian system, with its two moons (that likely used to be three), all show similar evidence of having been created by giant impacts long ago.
But now, scientists are noticing problems with the Giant Impact Hypothesis as originally formulated for creating Earth’s Moon. Instead, it looks like a smaller (but still very large) impact, from an object originating much farther out in our Solar System, may have been responsible for the creation of our Moon. Instead of what we call a giant impact, a high-energy collision with proto-Earth could have formed a debris disk around our world, creating a new type of structure known as a synestia.
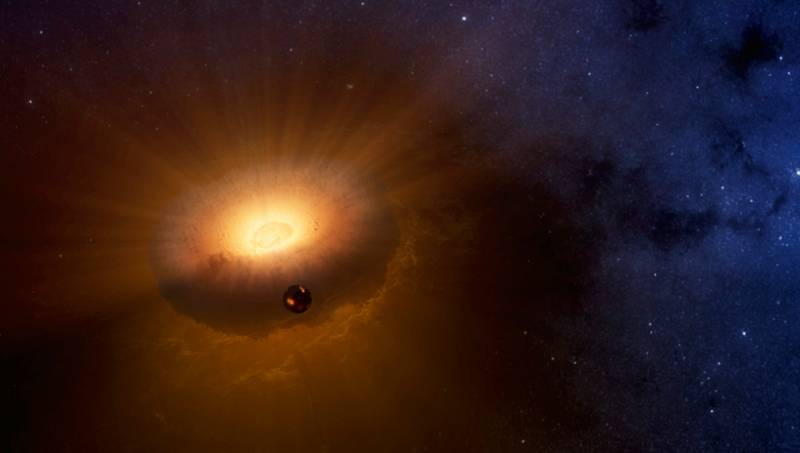
There are four big properties of our Moon that any successful theory for its origin must explain: why there is only one large moon rather than multiple moons, why the isotope ratios for elements are so similar between the Earth and Moon, why the moderately volatile elements are depleted in the Moon, and why the Moon is inclined as it is with respect to the Earth-Sun plane.
The isotope ratios are particularly interesting for the Giant Impact Hypothesis. The similar isotopic properties between the Earth and Moon suggest that the impactor (Theia) and Earth, if they were both large, had to be formed at the same radius from the Sun. This is possible, but models that form a Moon via that mechanism don’t give the right angular momentum properties. Similarly, grazing collisions with the right angular momentum give rise to different isotopic abundances than what we see.
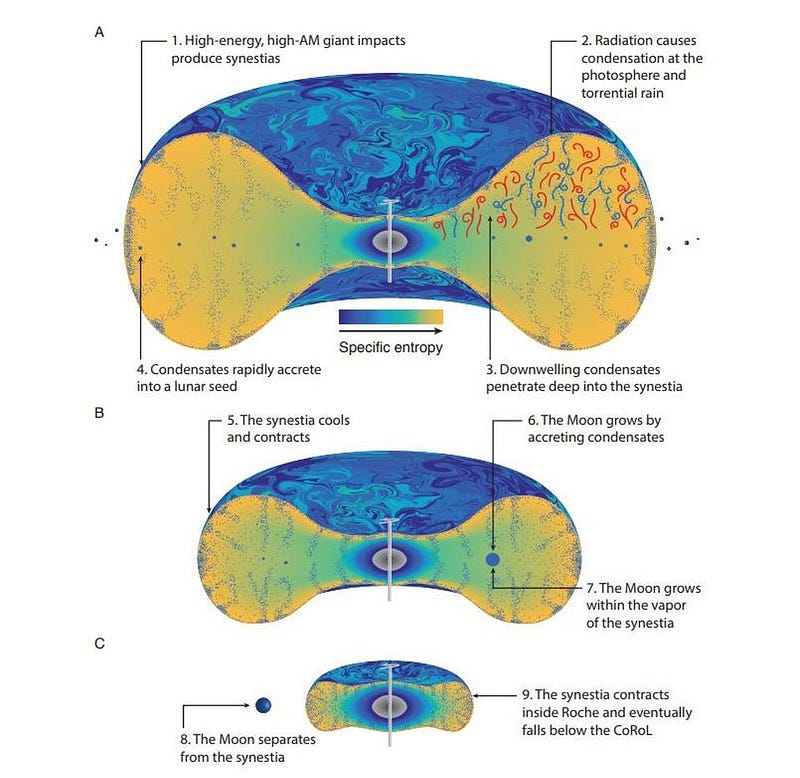
That’s why the alternative — a synestia — is so appealing. If you have a fast, energetic collision between a smaller body that’s less massive and our proto-Earth, you’d form a large torus-shaped structure around the Earth. This structure, called a synestia, is made of vaporized material that originated from a mix of proto-Earth and the impacting object.
Over time, these materials will mix, forming many mini-moons (called moonlets) in short order, which can stick together and gravitate, leading to the Moon we observe today. Meanwhile, the majority of the material in the synestia, particularly the inner part, will fall back to Earth. Rather than a single, contrived giant impact, we can now speak in terms of generalized structures and scenarios that give rise to large moons like our own.
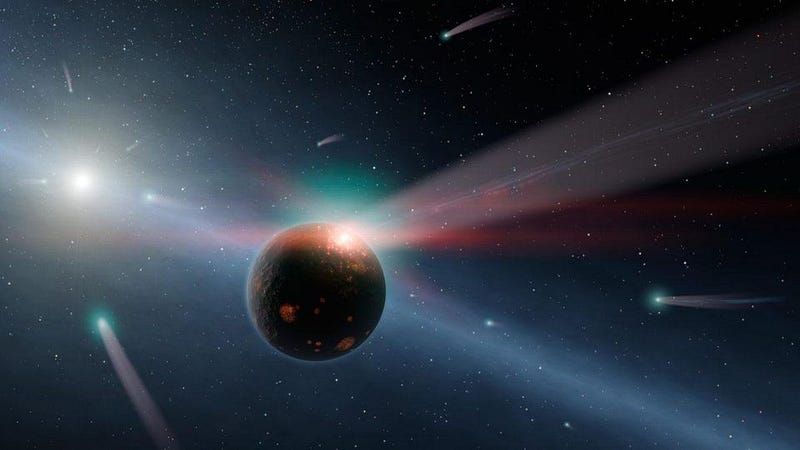
There was almost certainly a high-energy collision with a foreign, out-of-orbit object that struck our young Earth in the early stages of the Solar System, and that collision was required to give rise to our Moon. But it was very likely much smaller than Mars-sized, and it was almost certainly a sturdy strike, rather than a glancing collision. Instead of a cloud of rock fragments, the structure that formed was a new type of extended, vaporized disk known as a synestia. And over time, it settled down to form our Earth and Moon as we know them today.
At the end of the early stages of our Solar System, it was as promising as it could be for life. With a central star, three atmosphere-rich rocky worlds, the raw ingredients for life, and with gas giants only existing much further beyond, all the pieces were in place. We know we got lucky for humans to arise. But with this new understanding, we also think the possibility for life like us has happened millions of times before all throughout the Milky Way.
Further reading on what the Universe was like when:
- What was it like when the Universe was inflating?
- What was it like when the Big Bang first began?
- What was it like when the Universe was at its hottest?
- What was it like when the Universe first created more matter than antimatter?
- What was it like when the Higgs gave mass to the Universe?
- What was it like when we first made protons and neutrons?
- What was it like when we lost the last of our antimatter?
- What was it like when the Universe made its first elements?
- What was it like when the Universe first made atoms?
- What was it like when there were no stars in the Universe?
- What was it like when the first stars began illuminating the Universe?
- What was it like when the first stars died?
- What was it like when the Universe made its second generation of stars?
- What was it like when the Universe made the very first galaxies?
- What was it like when starlight first broke through the Universe’s neutral atoms?
- What was it like when the first supermassive black holes formed?
- What was it like when life in the Universe first became possible?
- What was it like when galaxies formed the greatest numbers of stars?
- What was it like when the first habitable planets formed?
- What was it like when the cosmic web took shape?
- What was it like when the Milky Way took shape?
- What was it like when dark energy first took over the Universe?
- What was it like when our Solar System first formed?