What The 3 Biggest Physics Discoveries Of The Decade Mean For The Future Of Science

Finding the Higgs boson, gravitational waves, and imaging a black hole’s event horizon were huge. There’s even more to the story.
From a scientific point of view, the 2010s have been a tremendously fruitful decade. Our knowledge of exoplanets — planets that orbit stars beyond our own — exploded, yielding thousands of new discoveries and an unparalleled understanding of what’s out there. The Planck satellite and our large-scale structure surveys pinned down dark energy, while improved astronomical data showed us a conundrum about the expanding Universe. Lasers got faster and more powerful; quantum supremacy was achieved for the first time; we explored Pluto and beyond, while our farthest spacecrafts at last entered interplanetary space.
But three physics advances stand head-and-shoulders above the rest, holding enormous ramifications for what the future of science holds. The discovery of the Higgs boson, the direct detection of gravitational waves, and the first image of a black hole’s event horizon revolutionized science in the 2010s, and will continue to influence physics for decades to come.
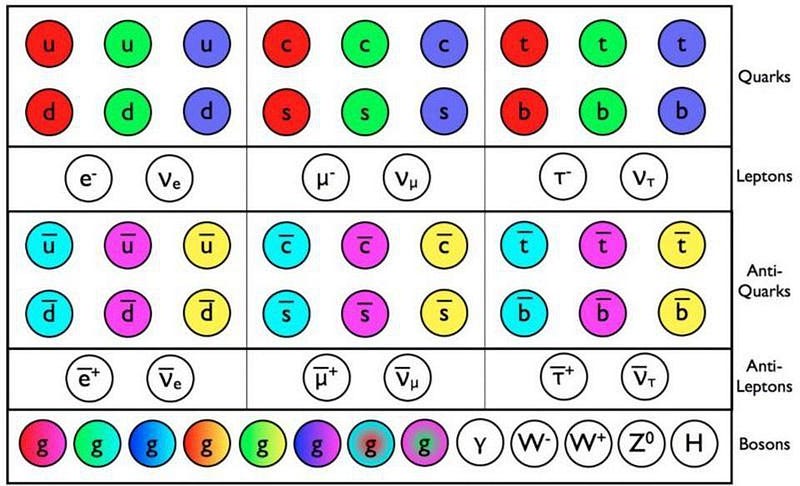
1.) Discovering the Higgs boson. With the quarks, charged leptons, neutrinos, and their antimatter counterparts already discovered prior to the 2010s, the fermionic sector of the Standard Model was already complete. We’d already discovered and measured the properties of all of the gauge bosons as well: the W and Z bosons, the gluons, and the photon. Only the Higgs boson — the last of the particles anticipated by the Standard Model — remained.
The Large Hadron Collider, the most powerful particle accelerator ever created by humanity, was constructed with the explicit goal of discovering this particle. By achieving energies never seen before in terrestrial accelerators and combining it with greater numbers of proton-proton collisions than ever before, scientists were able to at last reveal natures most elusive fundamental particle.
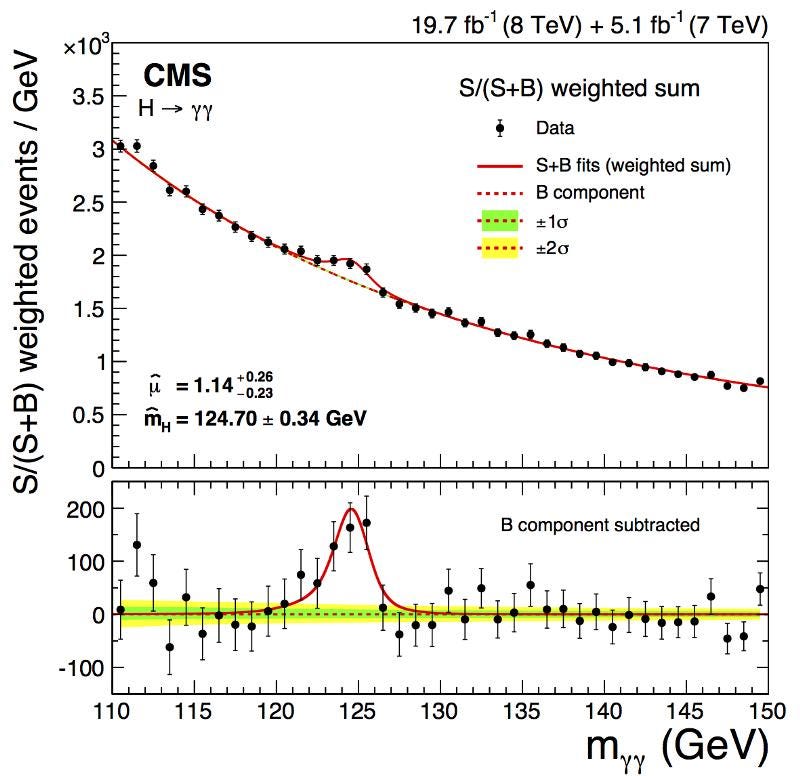
We were able to not only create and detect the Higgs, but we measured a number of its properties. These included:
- its mass, which has an equivalent energy of 125–126 GeV,
- its spin, which is zero, making it the only fundamental scalar particle ever seen,
- and its branching ratios, which show us how the Higgs boson is probabilistically likely to decay into various sets of particles.
In addition to discovering the Higgs, making these detailed measurements of these properties enabled us to compare theory with experiment, and to ask ourselves how successful the Standard Model was in predicting how the Higgs would behave. As of 2019 and the full suite of data that has been collected and analyzed by the CMS and ATLAS collaborations, everything we’ve seen is 100% consistent with the Higgs boson having the exact properties predicted theoretically.
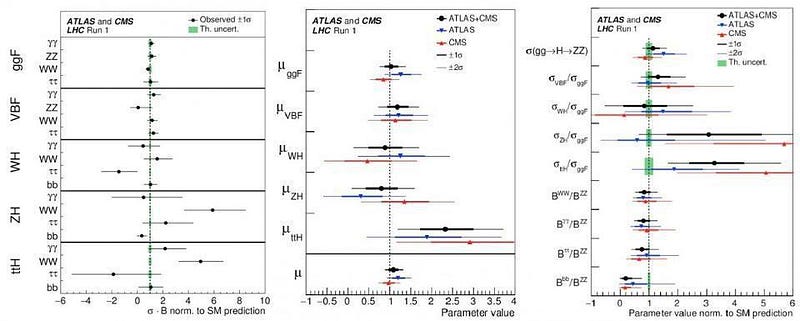
This itself is an enormous puzzle. On the one hand, we have a slew of mysteries about the Universe that the particles, fields, and interactions of the Standard Model cannot explain. We do not know the cause of dark matter, dark energy, inflation, or baryogenesis, only that the Standard Model alone cannot account for it. We have no solution to a myriad of other puzzles, from the strong CP problem to neutrino masses to explaining why particles have the rest masses that they do.
Scientists plan to run the Large Hadron Collider into the 2030s, performing a number of lower-energy experiments in parallel. But unless they reveal an answer or at least a compelling hint, humanity will face a controversial question: should we build a superior, next-generation collider to look beyond what the Large Hadron Collider can teach us? The future of particle physics — and a chance to finally unravel these mysteries — is at stake.
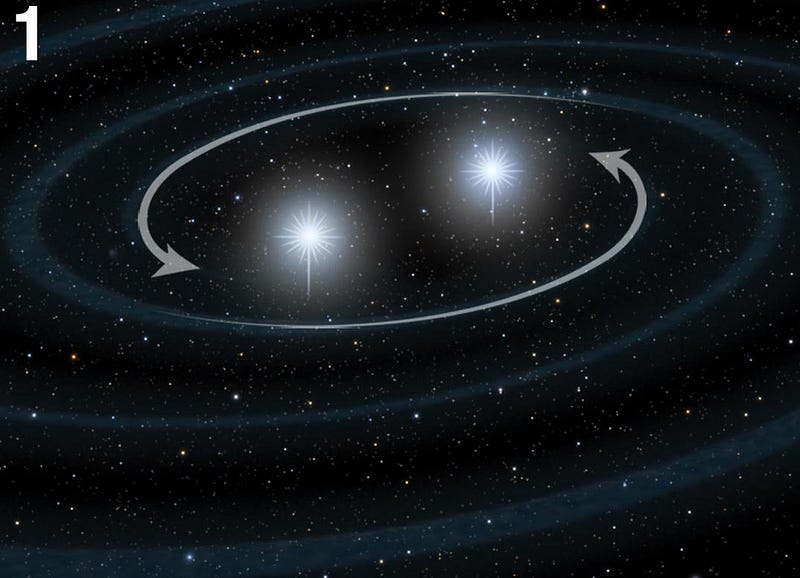
2.) The direct detection of gravitational waves. When Einstein put forth the theory of General Relativity in 1915, there were a whole slew of consequences that had not been sufficiently worked out within this paradigm-shifting new framework. After decades of theoretical work, however, it became clear that as masses moved through the Universe, the curvature of spacetime changed, and masses moving through a spacetime whose curvature changed with time needed to emit a new form of radiation: gravitational waves.
Although the indirect consequences of this radiation appeared in pulsar data long ago, the ultimate goal was always to detect these ripples directly. When a new generation of gravitational wave detectors came online in 2015, spearheaded by the LIGO collaboration, an entire new field was born: that of gravitational wave astronomy. For the first time, these ripples left observable, identifiable signals in human-created detectors, revealing their existence directly.
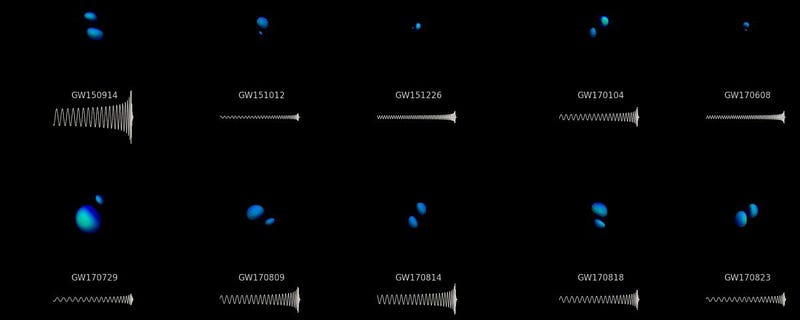
Two types of signals have already been seen directly: signals corresponding to the inspiral and merger of binary black holes, and signals corresponding to the merger of two neutron stars. The former is by far the most frequent type of signal that LIGO sees, revealing black holes in a mass range that had never been seen before and teaching us about the population statistics of these stellar remnants, while the latter comes along with electromagnetic signals, too, allowing us to determine the origin of the heaviest elements in the Universe.
Detectors such as LIGO and Virgo have already been upgraded, increasing their range and their sensitivity, and this current run may yet reveal not only new detections, but new classes of objects that generate gravitational waves, such as neutron star-black hole mergers, black holes of lighter masses than ever seen before, or possibly even pulsar quakes, supernovae, or something entirely surprising.
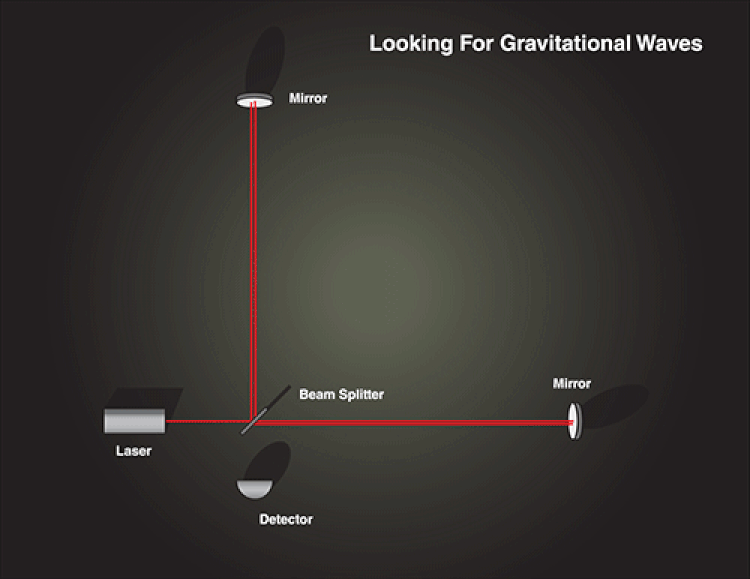
As the 2010s give way to the 2020s and beyond, gravitational wave detectors will continue to increase in size, sensitivity and scope, opening up the possibility of revealing signals we can only dream of detecting today. Objects falling into supermassive black holes are on our horizon, as are the gravitational waves generated during the final moments of inflation: the phase of the Universe that preceded and set up the hot Big Bang.
Up until very recently, humanity wasn’t even sure that gravitational waves existed. We weren’t sure these signals would show up in our instruments, or that our theoretical predictions would align with reality. The last four years have shown us that not only was Einstein right, but there’s an entire Universe out there to explore beyond the detection of electromagnetic (light) signals. This century promises to be the century of a new type of astronomy: gravitational wave astronomy. How far we go with it is entirely up to us.
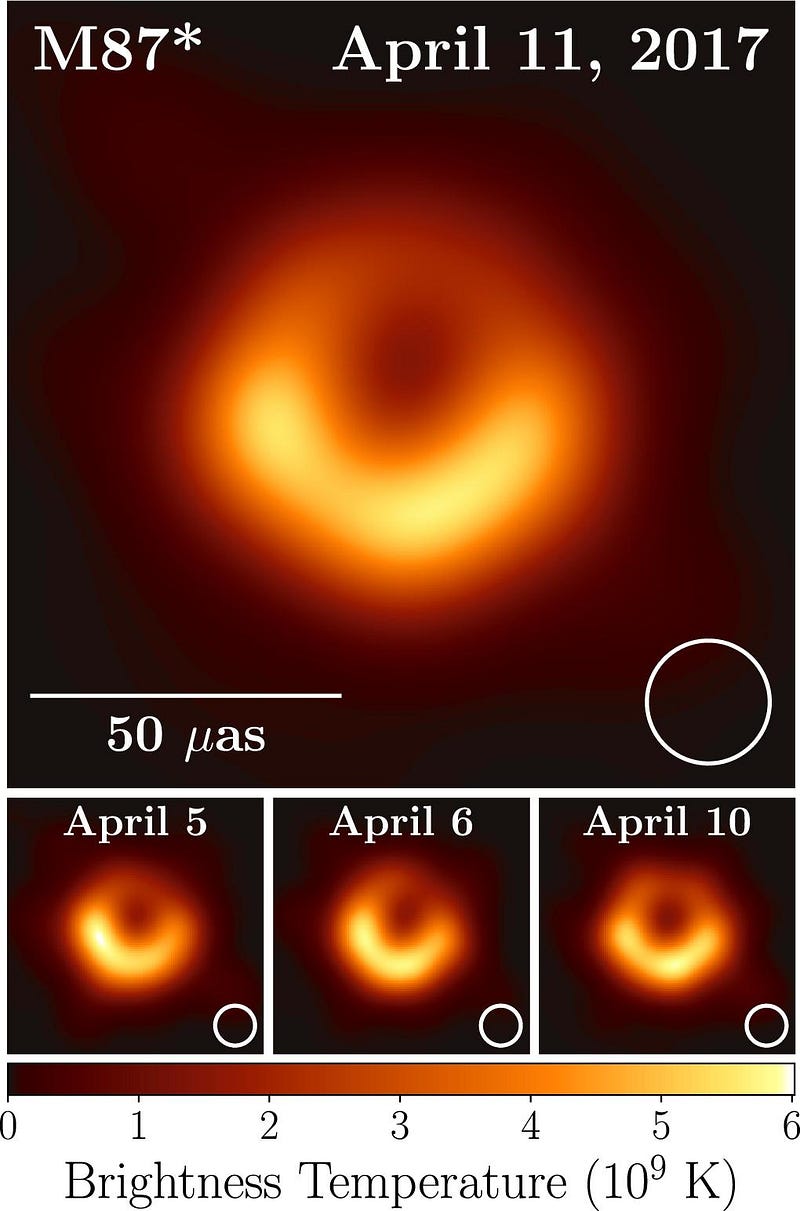
3.) Detecting the event horizon of a black hole directly. This achievement, the most recent of the three, dates back only to April of 2019, when the famed “donut” image of the supermassive black hole at the center of galaxy Messier 87 was released. Requiring hundreds of scientists using many petabytes of data collected simultaneously with radio telescopes and arrays of radio telescopes all across the world, this picture is just the tip of the iceberg.
Sure, it’s cool to see an event horizon for the first time, and to confirm yet another prediction of Einstein’s General Relativity. It’s an incredible technical achievement, leveraging a technique that has only become technically possible with the advent of new arrays such as ALMA. It’s remarkable that so many observatories were able to coordinate with one another, worldwide, to make these observations. But that’s not the biggest story.
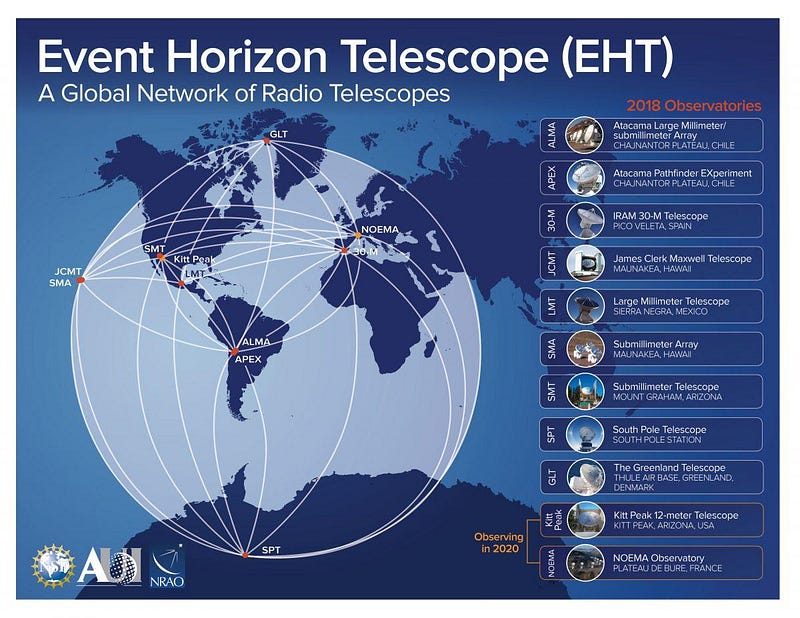
The most remarkable fact about all of this is that we are probing structures that are constantly changing with time down to precisions that were unimaginable a few years ago. The resolution of the Event Horizon Telescope is the equivalent of a single-dish telescope 12,000 kilometers in diameter: the size that a human fist on the Moon would appear to a human on Earth.
Much like the human fist example, the structures we’re observing are ones that are constantly changing, yet only observing a snapshot in time. The April 5/6 images of the black hole look similar to one another, but different from the April 10/11 images, demonstrating that the photons we’re observing are changing over time.
In the very near future, we expect to be able to tease out the signals of black hole flares, infalling matter, changes in the accretion flow, and maps of not just the radio light, but the polarization of that light. But in the more distant future, we can begin launching properly equipped radio telescopes into space, syncing them up with our ground-based observatories and extending the baseline (and hence, the resolution) of the Event Horizon Telescope to much greater precisions.
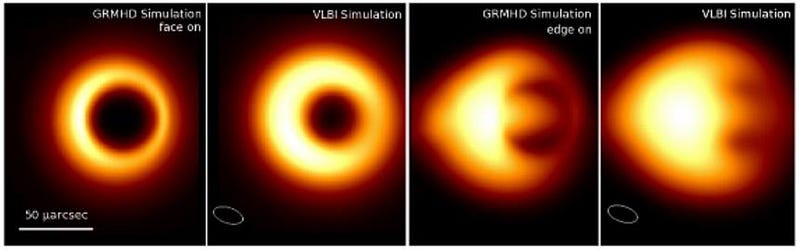
As the coming decades unfold, we won’t simply measure how one or two supermassive black holes in the Universe evolve, but dozens or even hundreds. It’s possible that stellar-mass black holes will enter the fold as well, as they’re contained within our own galaxy and thus appear relatively large. It’s even possible that we’ll get a surprise, and the black holes that appear to be quiet will exhibit radio signatures that these telescope arrays can pick up, after all.
There is a clear path laid out to continued exploration of the Universe, and all it relies on is extending what we’re already doing. We do not know what secrets nature holds beyond the already explored frontiers, but we do know one thing for certain: if we don’t look, we’ll never learn.
Ethan Siegel is the author of Beyond the Galaxy and Treknology. You can pre-order his third book, currently in development: the Encyclopaedia Cosmologica.