We Must Not Give Up On Answering The Biggest Scientific Questions Of All

Theoretical work tells you where to look, but only experiments can reveal what you’ll find.
There are fundamental mysteries out there about the nature of the Universe itself, and it’s our inherent curiosity about those unanswered questions that drives science forward. There’s an incredible amount we’ve learned already, and the successes of our two leading theories — the quantum field theory describing the Standard Model and General Relativity for gravity — is a testament to how far we’ve come in understanding reality itself.
Many people are pessimistic about our current attempts and future plans to try and solve the great cosmic mysteries that stymie us today. Our best hypotheses for new physics, including supersymmetry, extra dimensions, technicolor, string theory and more, have all failed to yield any experimental confirmation at all. But that doesn’t mean physics is in crisis. It means it’s working exactly as we’d expect: by telling the truth about the Universe. Our next steps will show us how well we’ve been listening.
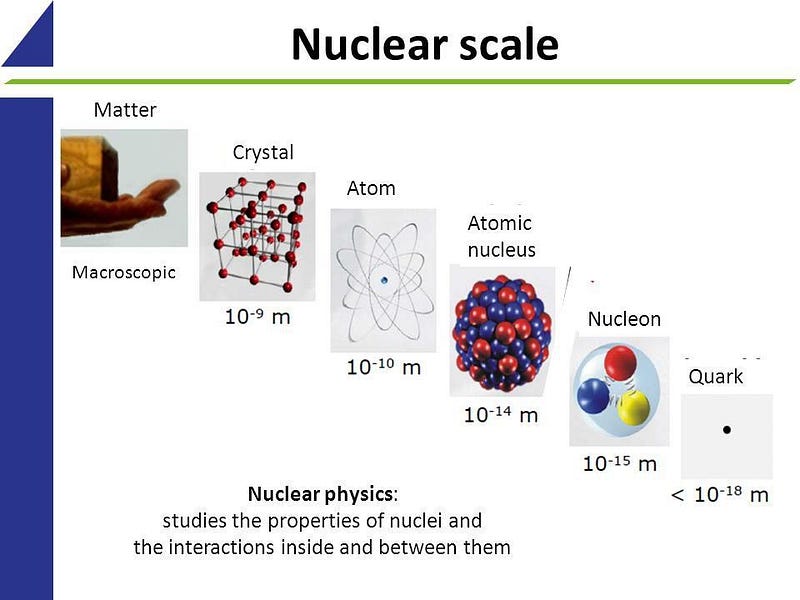
A century ago, the biggest questions we were able to ask included some enormous existential ones, such as:
- What are the smallest constituents of matter?
- Are our theories of the forces of nature truly fundamental, or is there a deeper understanding to be obtained?
- How big is the Universe?
- Has our Universe existed forever, or did it come into existence at some point in the past?
- How do the stars shine?
These were some of the biggest puzzles of their day, and were challenges that many didn’t think we’d be able to answer. In particular, they seemed to demand an investment of resources so enormous that there were calls to simply be content with what we knew at the time, and to simply use that knowledge to advance society.
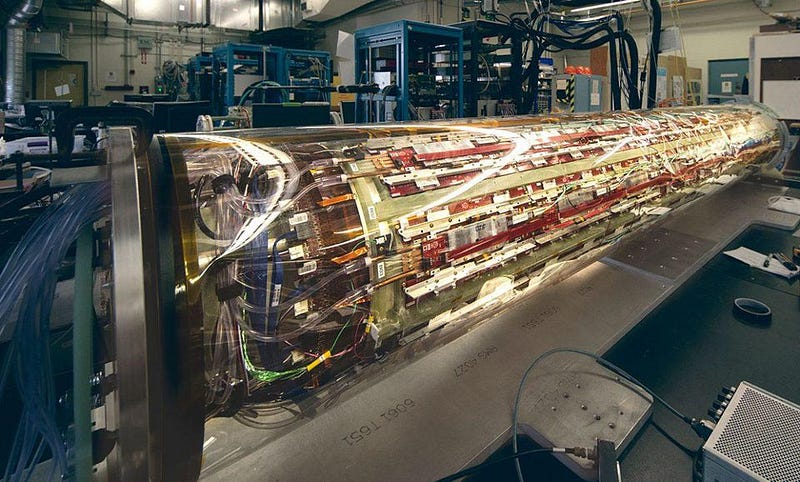
Of course, we did no such thing. Investing in society is tremendously important, but so is pushing the frontiers of what is known. With new discoveries and methods of inquiry, we were able to uncover the following answers:
- Atoms are made of subatomic particles, many of which have even smaller constituents; we now know of the entire Standard Model.
- Our classical theories were replaced by quantum ones, yielding four fundamental forces: the strong nuclear, electromagnetic, weak nuclear and gravitational forces.
- The observable Universe extends for 46.1 billion light years in all directions; the unobservable Universe may be much larger or even infinite.
- It has been 13.8 billion years since the event known as the hot Big Bang gave rise to the Universe we know, with an inflationary epoch of undetermined duration preceding it.
- And the stars shine based on the physics of nuclear fusion, converting matter into energy via Einstein’s E = mc².
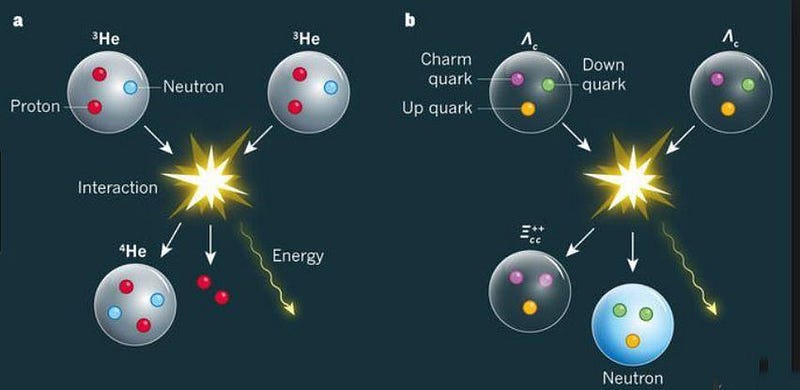
And yet, this only serves to deepen the scientific mysteries we have surrounding us. With everything we know about the fundamental particles, we know there should be more to the Universe than just the ones we know of. We cannot explain dark matter’s apparent existence, nor do we understand dark energy or why the Universe expands with the properties it does.
We do not know why the particles have the masses that they do, why matter dominates the Universe and not antimatter, or why neutrinos have mass at all. We do not know if the proton is stable or will someday decay, or whether gravity is an inherently quantum force in nature. And even though we know the Big Bang was preceded by inflation, we do not know whether inflation itself had a beginning, or was eternal to the past.
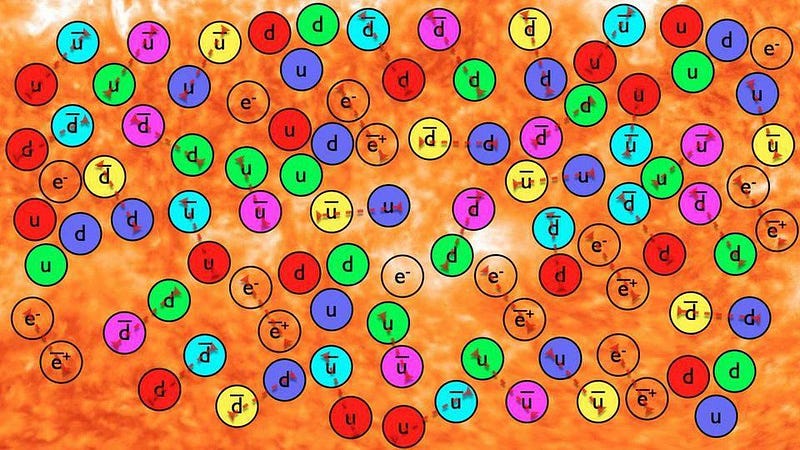
Are these mysteries currently solvable by human beings? Can the experiments we’re capable of performing with either current or near-future technology shed any light on these fundamental puzzles?
The answer to that first question is perhaps; we do not know what secrets nature holds unless we look. The answer to that second question, however, is an unambiguous yes. Even if every theory we’ve ever theorized for what lies beyond the present frontier of what is known — the Standard Model and General Relativity — is 100% wrong, there is an astounding amount of information to be gained by performing the experiments we’re designing for the next generation. To not build them would be an enormous folly, even if it merely confirms the nightmare scenario that particle physicists have been fearing for generations.
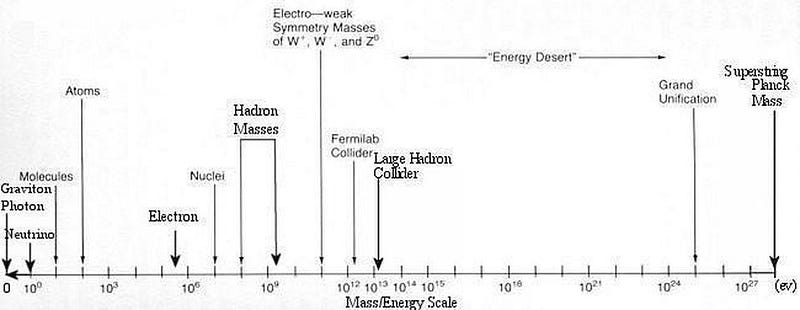
When you hear about a particle accelerator, you probably think about all the new discoveries that might await us at higher energies. The promise of new particles, new forces, new interactions, or even entirely new sectors of physics are what theorists frequently concoct and promote, even as experiment after experiment fails to deliver on those promises.
There’s a good reason for this: most of the ideas one can concoct in physics have already been either ruled out or highly constrained by the data we already have in our coffers. If you want to discover a new particle, field, interaction, or phenomenon, it doesn’t do you any good to postulate something that’s inconsistent with what we already know to be true today. Sure, there might be assumptions we’ve made that later turn out to be incorrect, but the data itself must be in agreement with any new theory.
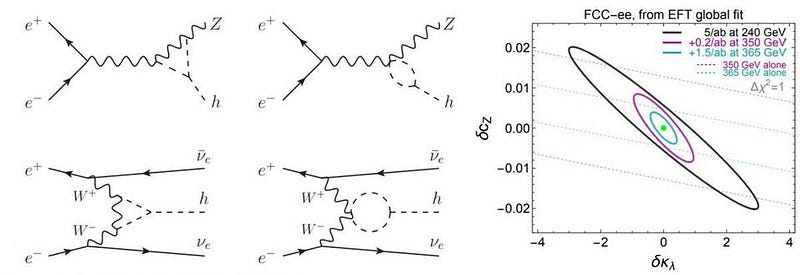
That’s why the greatest amount of effort in physics goes not into new theories or new ideas, but into experiments that push past the regimes we’ve already explored. Sure, finding the Higgs boson may make tremendous headlines, but how strongly does the Higgs couple to the Z-boson? What are all the couplings between those two particles and the others in the Standard Model? How easy are they to create? And once you create them, are there any mutual decays that are different from a standard Higgs decay plus a standard Z-boson decay?
There’s a technique you can use to probe this: create an electron-positron collision at exactly the mass of the Higgs plus the Z-boson. Instead of a few dozen to perhaps 100 events that create both a Higgs and a Z-boson, which is what the LHC has yielded, you can create thousands, hundreds of thousands, or even millions.
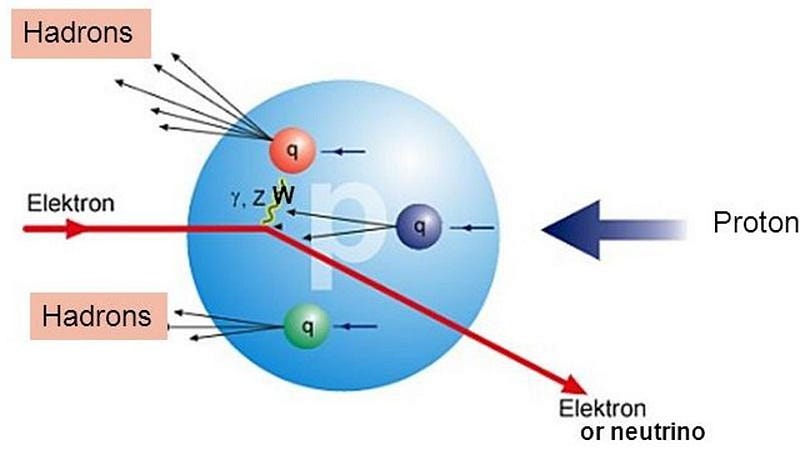
Sure, the general public might get more excited about a brand new particle than anything else, but not every experiment is designed to make new particles, nor should they be. Some are designed to probe matter that we already know exists, and to study its properties in detail as never before. LEP, the Large Electron-Positron collider and the predecessor to the LHC, never found a single new fundamental particle. Neither did the DESY experiment, which collided electrons with protons. Neither did RHIC, the Relativistic Heavy Ion Collider.
And that’s to be expected; that wasn’t the point of those colliders. Their purpose was to study the matter that we know exists to never-before-studied precisions.
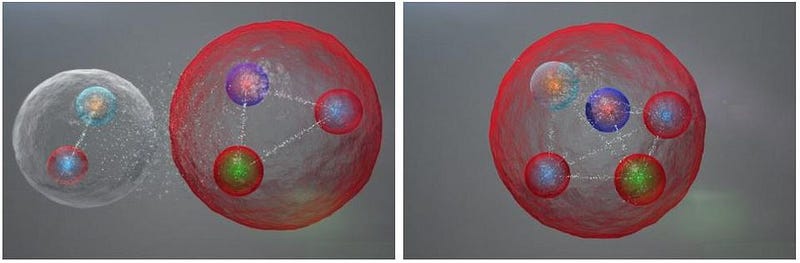
It isn’t as though these experiments simply confirmed the Standard Model, although everything they discovered was consistent with the Standard Model and nothing more. They created new composite particles, and measured couplings between them. Decay ratios and branching ratios were discovered, as were subtle differences between matter and antimatter. Some particles were discovered to behave differently from their mirror-image particles. Others were found to violate time-reversal symmetry. Still others were found to mix together, creating bound states that we never realized could exist before.
The purpose of the next great science experiment isn’t to simply look for one new thing or test one new theory. It’s to gather a huge suite of otherwise unattainable data, and to let that data guide the development of the field.
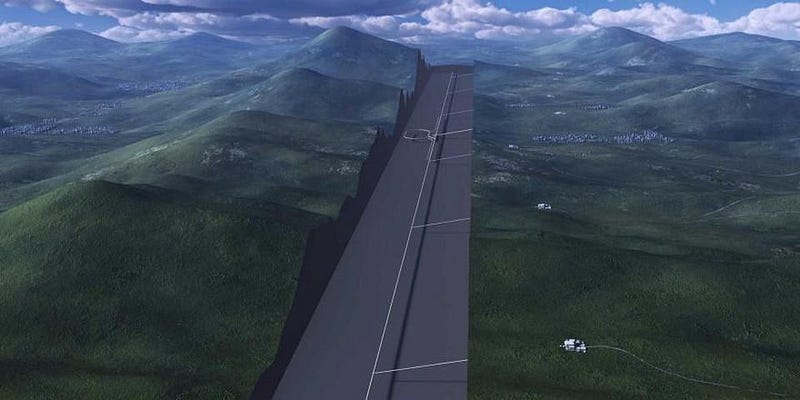
Sure, we can design and build experiments or observatories with an eye towards what we anticipate might be there. But the best bet for the future of science is a multi-purpose machine that can gather large and varied amounts of data that could never be collected without such a tremendous investment. It’s why Hubble was so successful, why Fermilab and the LHC have pushed boundaries as never before, and why future missions such as the James Webb Space Telescope, future 30-meter class observatories like the GMT or the ELT, or future colliders beyond the LHC such as the FCC, CLIC, or the ILC are required if we ever hope to answer the most fundamental questions of all.
There’s an old saying in business that applies to science just as well: “Faster. Better. Cheaper. Pick two.” The world is moving faster than ever before. If we start pinching pennies and don’t invest in “better,” it’s tantamount to already having given up.
Ethan Siegel is the author of Beyond the Galaxy and Treknology. You can pre-order his third book, currently in development: the Encyclopaedia Cosmologica.