Throwback Thursday: The Big Bang’s Last Great Prediction
Every prediction it’s ever made has been verified, except for one.
“These neutrino observations are so exciting and significant that I think we’re about to see the birth of an entirely new branch of astronomy: neutrino astronomy.” -John Bahcall
If you’ve been coming around here at all over the past six years and counting, you know about the Big Bang. Yes, the vast majority of galaxies that we know of are speeding away from us, but there’s more than that; on average, the farther away each individual one is from us, the faster it appears to be receding.
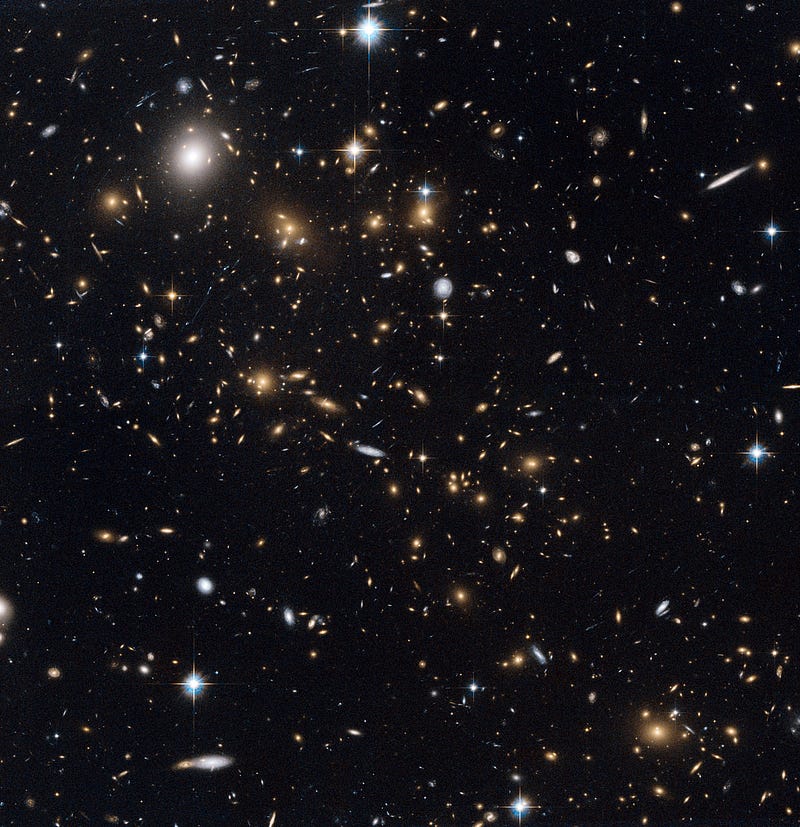
When we look across those great distances to those galaxies moving at fantastic speeds, we’re also looking at the Universe when it was different than it is today. Because the speed of light is finite, you’re actually looking at these galaxies as they existed in the distant past. Since all the galaxies are expanding away from one another, and galaxies that are farther away are expanding away at a faster rate, this led to the idea that the Universe was smaller, denser, and also hotter in the past.
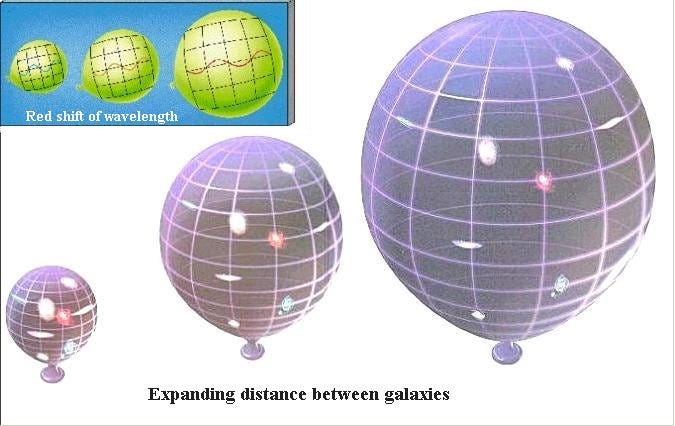
Going backwards in time, because the Universe was hotter, it was once so hot that neutral atoms couldn’t even form: everything was a sea of ionized plasma, filled with nuclei, electrons, and radiation. (When the Universe cooled to form neutral atoms, that’s where the cosmic microwave background comes from.)
Going even farther back, you can imagine a Universe so hot that even the atomic nuclei can’t hold together against the intense bath of radiation; a high-enough energy photon will blast them apart into free protons and neutrons.
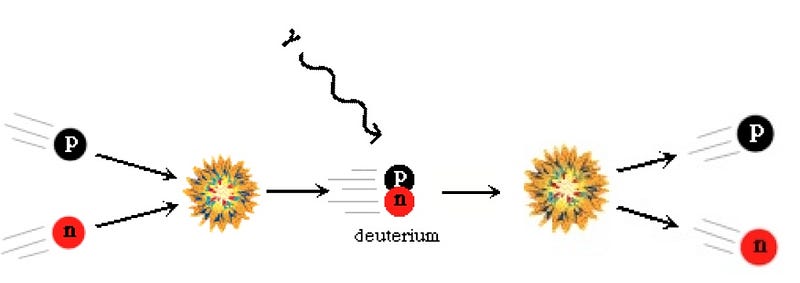
It was, in fact, when that era ended, and the Universe cooled enough that photons couldn’t blast those nuclei apart, that we started forming heavier elements for the first time in the Universe’s history; that leftover signature is another one of the great confirmations of the Big Bang.
But going even farther back than that, we can find a time where the radiation in the Universe was so hot that all the particles that exist, along with their antiparticles, would be spontaneously created in particle-antiparticle pairs due to these unavoidable high-energy collisions.
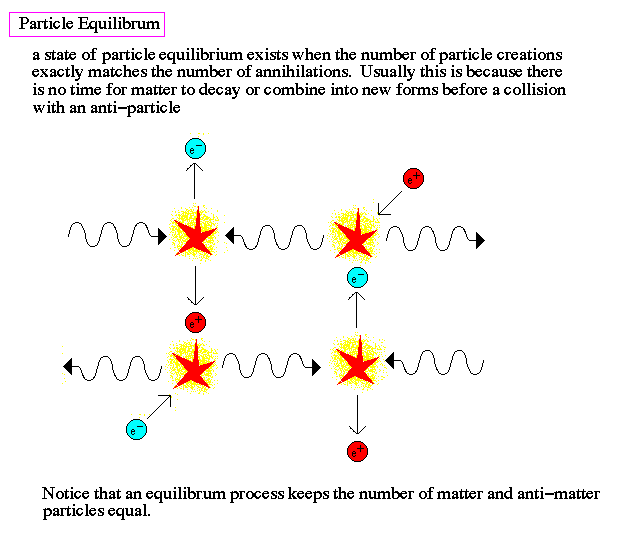
This includes all the quark/antiquark pairs, all the lepton/antilepton pairs, all the gluons and photons and the weak bosons, even the Higgs, and any additional, heretofore undiscovered particles that might exist at even higher energies than we currently understand. Back when the entire observable Universe — now nearly 100 billion light-years in diameter — was compressed into a space smaller than a single light-year across, these particle/antiparticle pairs all existed in great abundance, spontaneously creating and annihilating in an (approximately) equilibrium state.
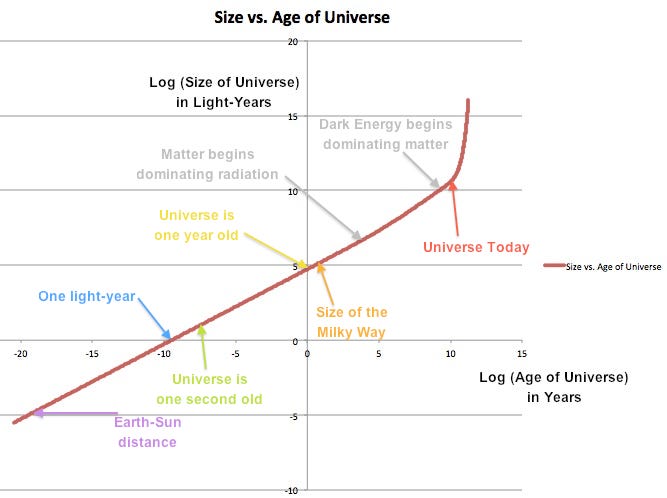
The amount of time that the Universe was in this state was very short — less than a second — but at these densities and energies, the interaction rate is more than large enough to have all of this happen spontaneously.
But — as you can clearly see — that equilibrium state doesn’t last for very long. As the Universe expands, it also cools (and hence its temperature drops), and it becomes harder and harder to make new particle-antiparticle pairs. Meanwhile, the existing ones will continue to annihilate away into photons, or particles of light. Eventually, the chance of annihilating — dependent on their cross-section — will drop to such a low value that whatever exists at that time will be effectively “frozen in,” and as long as that particle is stable against decay, it will continue to exist to the present day.
We know of three such species of particles (and their antiparticles) that do this: the neutrinos!
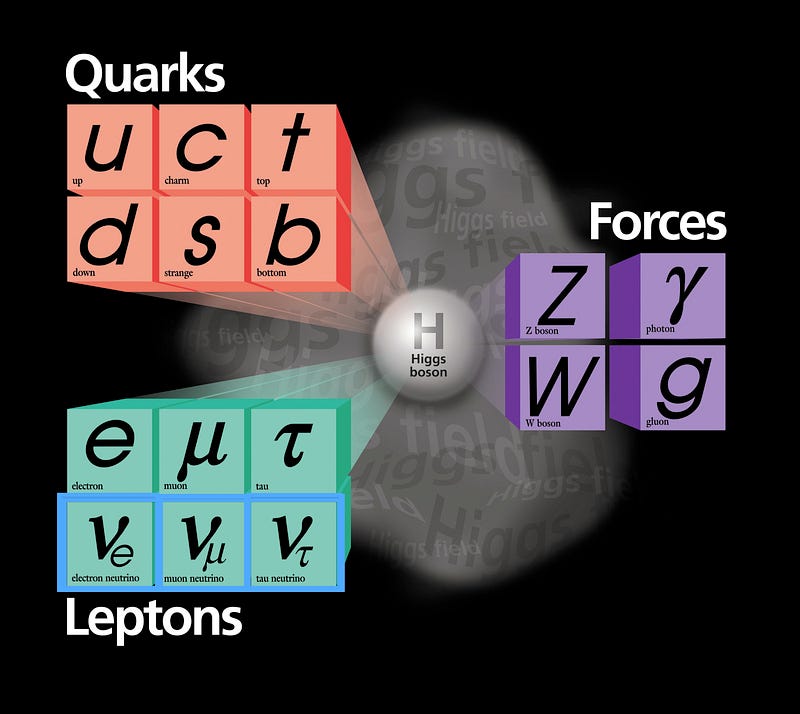
Coming in three flavors to match the three types of lepton — electron, muon and tau — these are the lightest, lowest-mass particles known to actually have a non-zero mass. The upper limit on the mass of the heaviest neutrino is still more than 4 million times lighter than the electron, the next lightest particle.
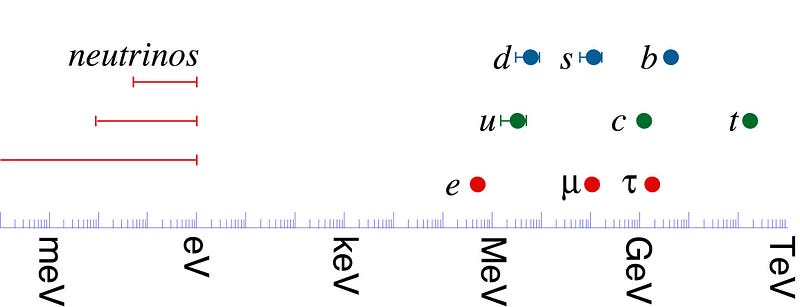
And yet, neutrinos have an energy-dependent cross section that becomes extremely small at lower energies. By time the Universe is about a single second old, the neutrinos and anti-neutrinos stop interacting with one another, and simply continue to lose energy and cool with the expansion of the Universe. You may remember that this is the same thing that photons do once neutral atoms are formed, which is where the cosmic microwave background comes from.
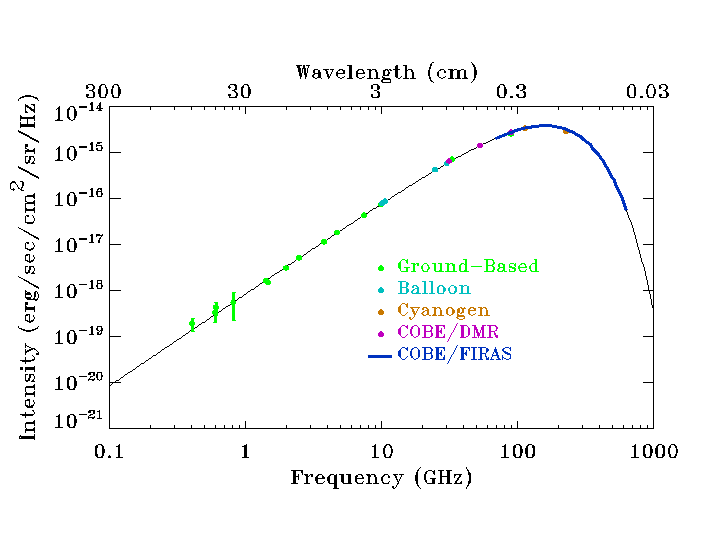
Only, neutrinos are slightly different than photons. Even though they have the tiniest masses of anything we know, because we know where they come from (and what the Universe was like when they stopped interacting), we know they don’t do exactly the same thing. The cosmic microwave background (CMB) of photons has an energy spectrum like the one above, with a peak at a temperature of 2.725 Kelvin.
The cosmic neutrino background should have a slightly lower temperature at 1.96 Kelvin (because electrons/positrons hadn’t annihilated yet; that’s why the CMB is slightly hotter), and there should be slightly fewer of them than there are photons; about 82% as many. (336 per cubic centimeter, with all three species and antineutrinos included, too, as compared to 411 per cubic centimeter for photons.) But remember, there’s one incredibly important difference between the cosmic microwave background and the cosmic neutrino background: unlike photons, neutrinos have a rest mass!
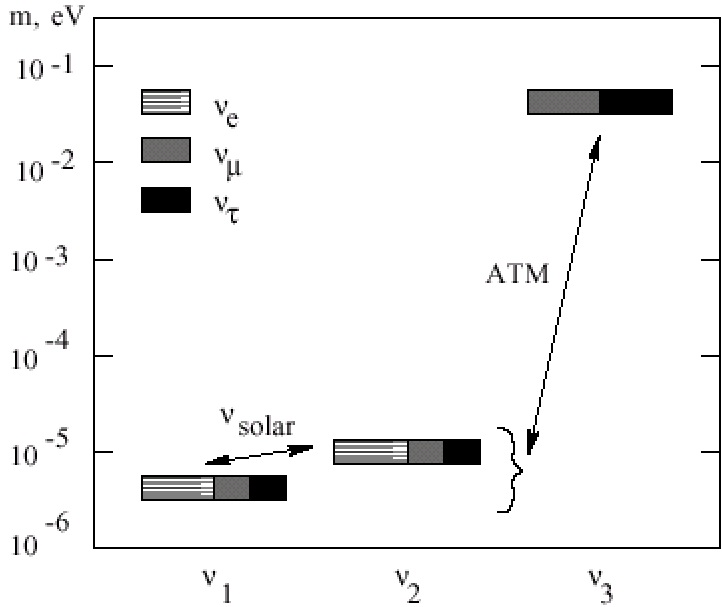
That mass, tiny though it may be, is still large compared to the amount of energy that corresponds to the thermal energy that’s left over from the early Universe. Depending on their mass (remember, there’s still some uncertainty), they’re moving at no more than a few thousand km/s today, and probably at just a few hundred km/s.
And this is a really, really interesting number.
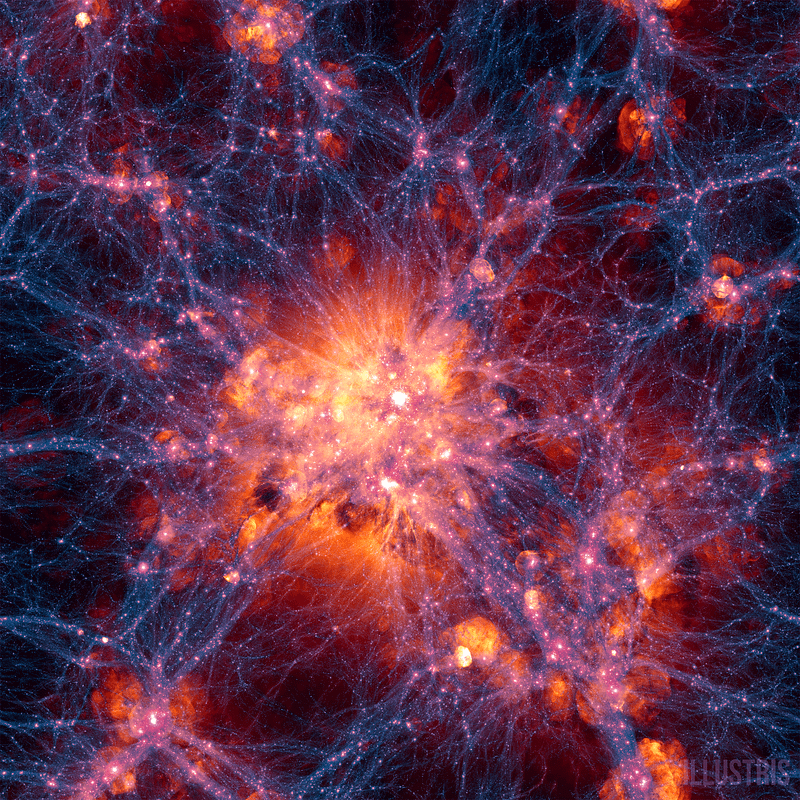
The mass-and-energy of these neutrinos tell us that they’ve fallen into the large-and-small-scale structures in the Universe, including in our own galaxy. They tell us that they’re a small percentage of the dark matter — between about 0.5%-and-1.4% of it — but cannot be all of it. There’s about as much mass in neutrinos as there is mass in the form of stars currently burning through their fuel today. Not a lot, but still interesting!

But what’s maybe most amazing about these neutrinos is that we have no practical idea about how we could experimentally detect them!
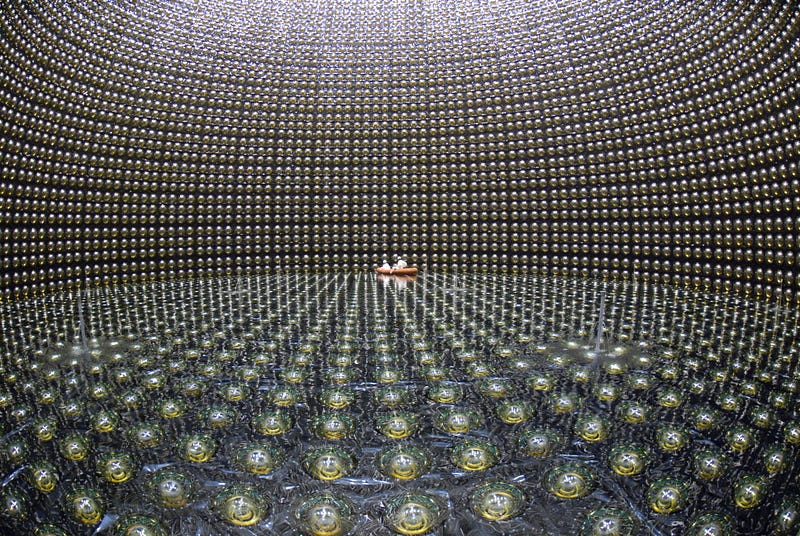
We can detect neutrinos, but only neutrinos with about a billion times the energy of these cosmic relics. Because of how quickly (exponentially) the cross-section falls off, we really have no hope for how to detect something with such a small signature; all of the neutrino detectors we’ve built and successfully implemented rely on ultra-high-energy neutrinos.
So our proven neutrino detection techniques wouldn’t be applicable unless you took a giant neutrino detector like Super-Kamiokande, above (or IceCube, at the very top), and accelerated the entire thing to relativistic speeds. Then — and only then — could you begin to get a signal akin to the one we get from the abundant, high-energy neutrinos that are easy to detect: the ones from the Sun and from nuclear reactors.
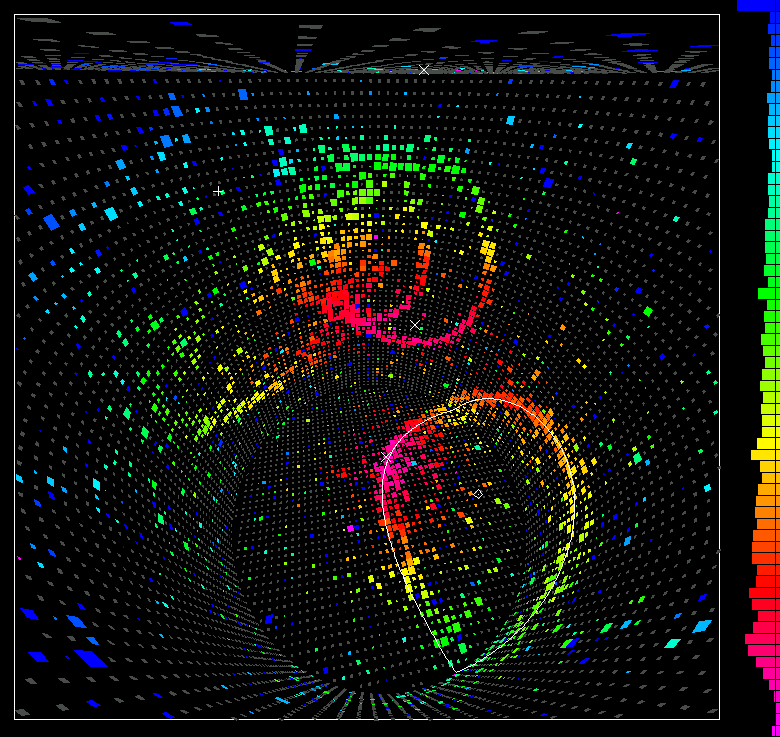
Since that’s impractical, to say the least, this is one of the last great untested predictions of the Big Bang, and one we’re unlikely to solve anytime soon. (If the gravitational waves from inflation do, in fact, hold up, this may be the final unverified prediction of the Big Bang!) Despite the fact that there are hundreds of these neutrinos and antineutrinos per cubic centimeter, and despite the fact that they’re zipping around at (at least) hundreds of kilometers per second, the only interaction they can conceivably have with normal matter is via a nuclear recoil.
And a nucleus, compared to a neutrino, is large, to put it mildly. Detecting one of these recoils is more difficult than detecting the recoil of a supremely-heavily-loaded semi-truck when it collides with… a paramecium. In other words, even if we could detect it, being able to discern an “event” from the experimental noise is well beyond our practical capabilities.
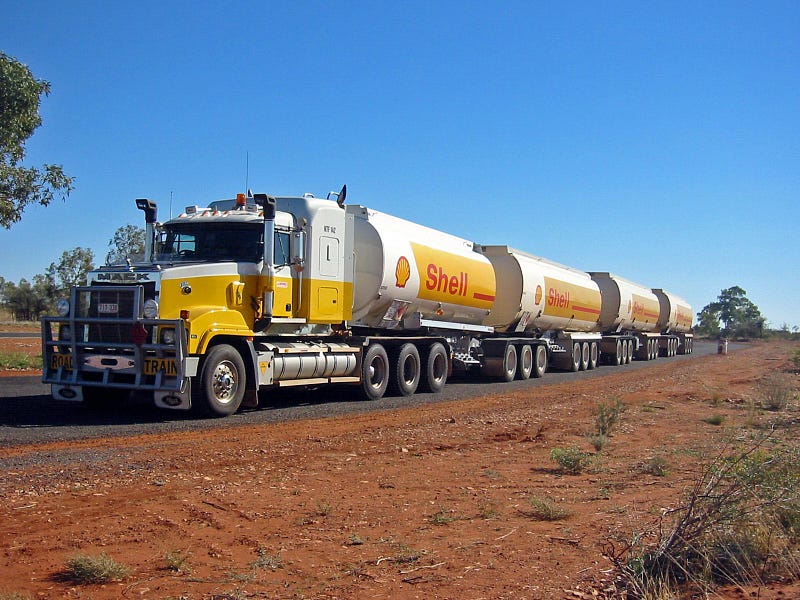
But there is one interesting thing we’ve learned about these neutrinos. You see, we’ve known for a long time that neutrinos are all left-handed, which is to say that their spin always opposes their momentum, or that they’re spin -½. On the other hand, anti-neutrinos are all right handed, their spin always points in the same direction as their momentum, or that they’re spin +½. All the other particles of half-integer-spin we know of have versions that are ±½, whether they’re matter or antimatter.
But not neutrinos. It’s fueled speculation that neutrinos might actually be their own antiparticles, making them a special type of particle known as a Majorana Fermion. But there’s a special type of decay that should happen if they are; so far, no dice on that decay, and because of that, the window on neutrinos being Majorana particles is closing.
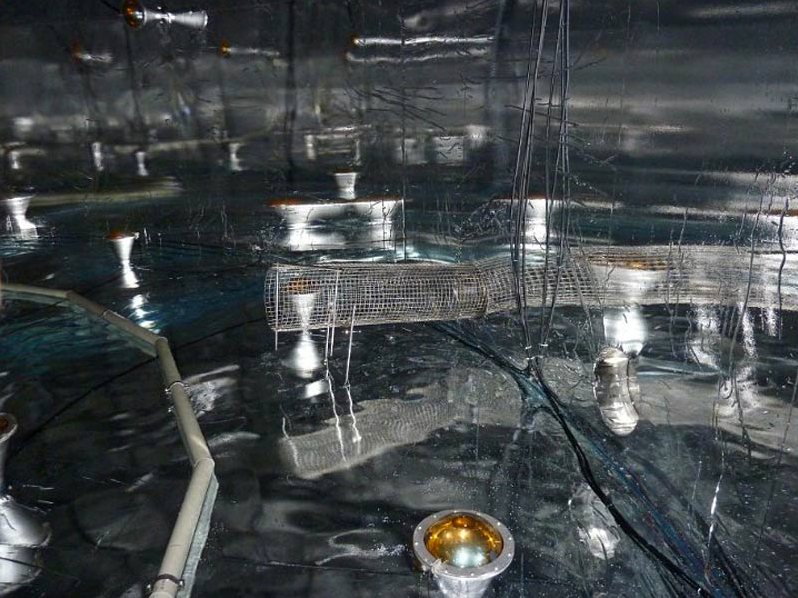
So there you have it: there are some 10^90 neutrinos and anti-neutrinos left over from the Big Bang, making them the second most abundant particle in the Universe (after photons). There are more than a billion ancient neutrinos out there for each proton in the Universe. And yet, all of these relic neutrinos — making up the cosmic neutrino background (or CNB) — are completely undetectable to us. Not in principle, just in practice, as we don’t know how to make experiments sensitive enough (or even close) to search for this, or to tease out such a signal against an overwhelming background of events. If you want to know what you can do to win a Nobel Prize, come up with a way to detect them, and the medal and the glory will surely be yours!
Until then, all we can do is marvel at what’s perhaps the last great unverified prediction of the Big Bang: a relic background of cosmic neutrinos!
Have a suggestion on how to win that Nobel? Tell us at the Starts With A Bang forum on Scienceblogs!