This One ‘Anomaly’ Is Driving Physicists To Search For Light Dark Matter
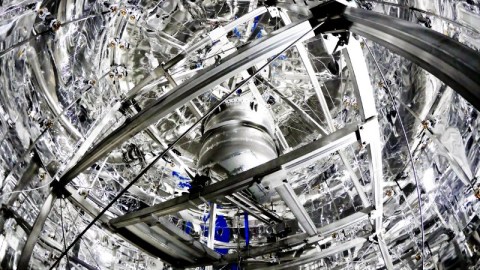
When you’re trying to peel back the veil obscuring the fundamental nature of matter, you have to look absolutely everywhere.
Sometimes, the solution to a puzzle you’ve been stymied by lies in a place you’ve already looked. Only, until you develop better-precision tools than you’ve used to conduct your previous searches, you won’t be able to find it. This has played out many times in the sciences, from the discovery of new particles to uncovering phenomena like radioactivity, gravitational waves, or dark matter and dark energy.
We’ve been looking for new particles not predicted by the Standard Model with an enormous variety of experiments for decades, from accelerators to underground laboratories to rare, exotic decays of everyday particles. Despite decades of searching, no beyond-the-Standard-Model particles have ever turned up. But recently, searches have begun to consider light dark matter, despite having already looked in that expected range. We have to look better, and one unexplained experimental result is the reason why.
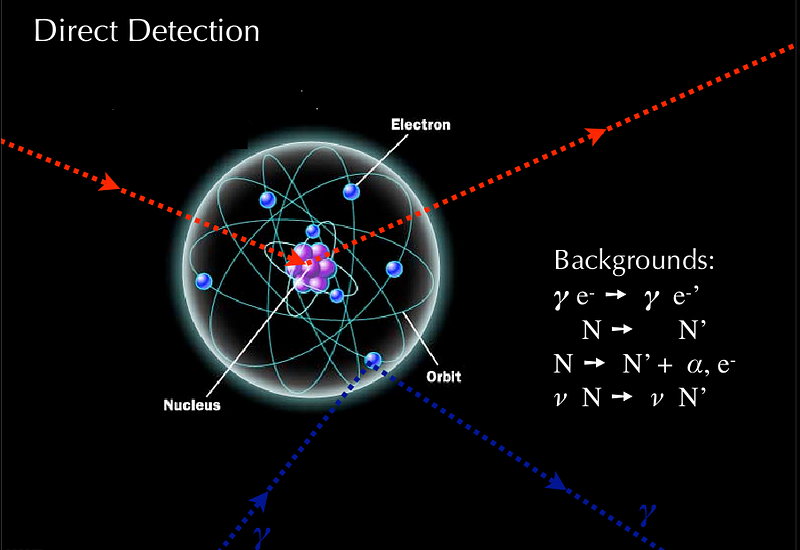
Identifying a scientific puzzle — a phenomenon or observation that cannot be conventionally explained — is often the starting point that leads to a scientific revolution. If heavy elements are made from the synthesis of lighter ones, for example, then you have to have a viable pathway for the natural construction of the heavy elements we see today. If your best theory cannot explain why carbon exists, but we observe carbon to exist, that’s a good puzzle for science to investigate.
Often, the puzzle itself offers possible clues to a solution. The fact that there are no stationary, oscillating in-phase electric and magnetic fields led to Special Relativity. If not for a mysterious observation of missing energy in radioactive beta decays, we wouldn’t have predicted the neutrino. And patterns seen in the heavy composite particles produced in accelerators led to the quark model and the prediction of the Ω- baryon.
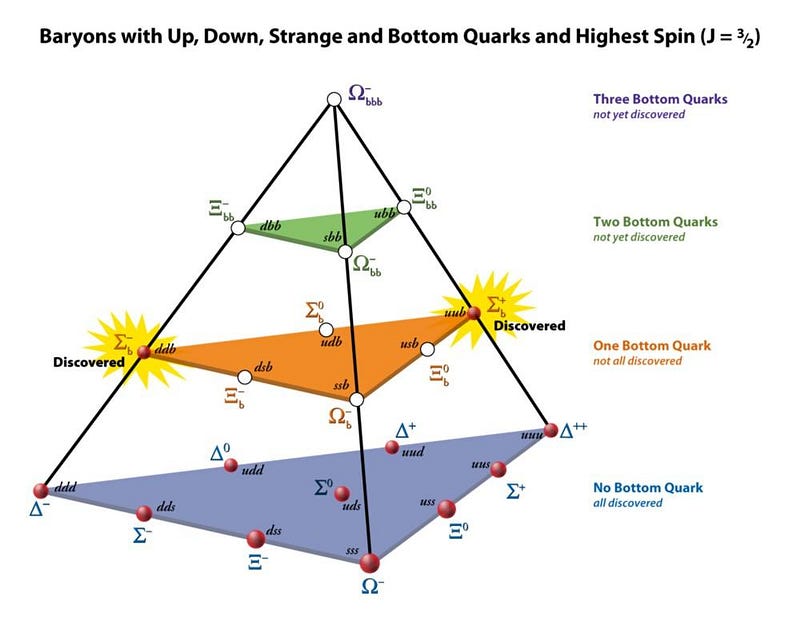
In the case of the mystery of carbon’s existence, the situation has only gotten more interesting with time. Back in the 1950s, scientist Fred Hoyle, along with Geoffrey and Margaret Burbidge, were trying to understand how the heavier elements of the periodic table were formed if all you began with were the lightest ones of all.
Postulating that the Sun was powered by the energy released from the nuclear fusion of light elements into heavy ones, Hoyle could account for the synthesis of deuterium, tritium, helium-3 and helium-4 from raw hydrogen nuclei (protons), but couldn’t find a way to get to carbon. You couldn’t add a proton or neutron to helium-4, since both helium-5 and lithium-5 were unstable: they’d decay after ~10^-22 seconds. You couldn’t add two helium-4 nuclei together, because beryllium-8 was too unstable, decaying after ~10^-16 seconds.
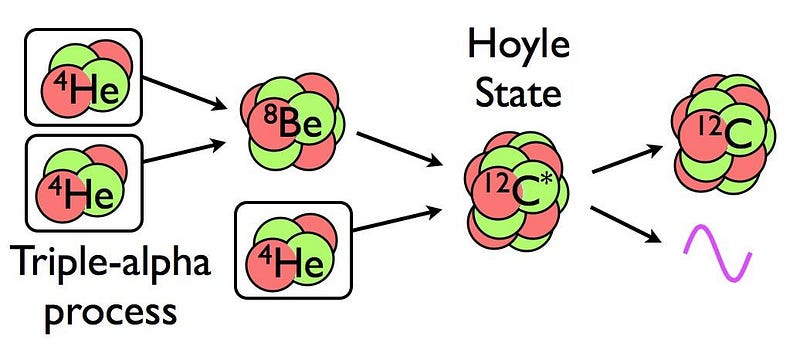
But Hoyle had a brilliant possible solution up his sleeve. If a dense enough environment could create beryllium-8 on fast enough timescales, it could be possible for a third nucleus — another helium-4 — to get in there before the beryllium decayed away. Mathematically, that would enable you to create carbon-12: permitting the existence of carbon under the right conditions.
Unfortunately, we knew the mass of a carbon-12 nucleus, and it didn’t match the mass of helium-4 plus the mass of beryllium-8. Unless our understanding of nuclear physics was wrong, this reaction couldn’t account for the carbon we see today. But Hoyle’s workaround was brilliant: he hypothesized the existence of another, hitherto undiscovered possibility: a resonant state of carbon-12 could exist that did have the right mass.
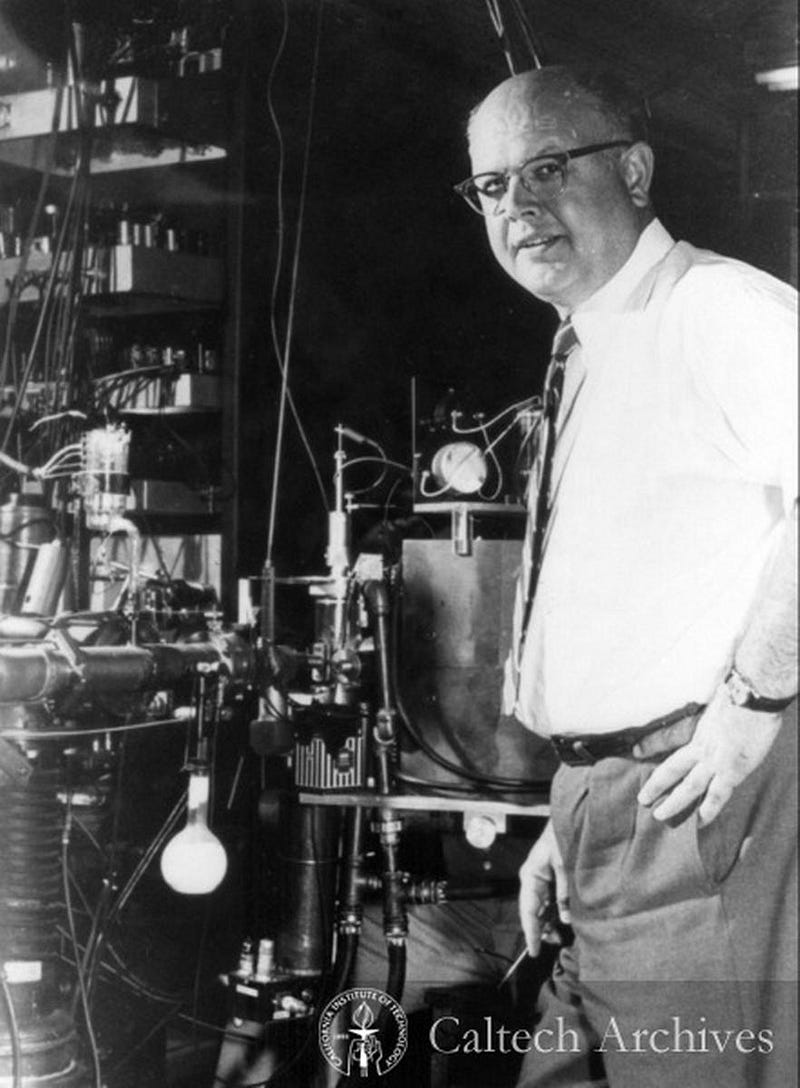
Then, it could decay to the carbon-12 we see today. This nuclear process, the triple-alpha process, is now known to occur inside red giant stars, with the resonant state of carbon-12 now known as the Hoyle state, as it was confirmed by nuclear physicist Willie Fowler later in the 1950s. The existence of carbon, and the puzzle of how to create it using known physics and pre-existing ingredients, led to this remarkable discovery.
Perhaps, then, a similar line of reasoning could lead to a solution to the biggest puzzles facing physicists today?
It’s undoubtedly worth a try. We all know that these big puzzles include dark matter, dark energy, the origin of the matter/antimatter asymmetry in our Universe, the origin of neutrino mass and the incredible difference between the Planck scale and the actual masses of the known particles.
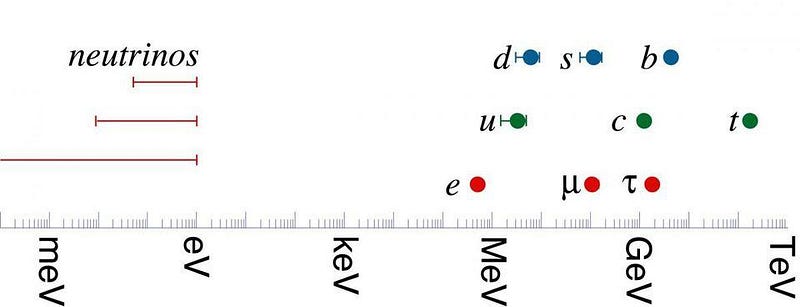
On the other hand, we have clues from measurements and observations that our current story of the Universe may not be all that there is. Most of these have not yet reached the definitive 5-sigma threshold we require to claim that something new is out there, but they are suggestive.
- The muon’s measured magnetic moment doesn’t match theoretical predictions with a 3.6-sigma tension.
- The AMS experiment has seen an excess of positrons, with an energy cutoff seen with 4.0-sigma confidence.
- And the tension between different methods of measuring the Hubble expansion rate has risen to a 4.4-sigma discrepancy.
But one experiment blew past that threshold years ago: an experiment designed to measure the decay of that short-lived state so essential to creating carbon in the Universe: beryllium-8. It disagrees with our conventional predictions by an impressive 6.8-sigma, and is known in the community as the Atomki anomaly.
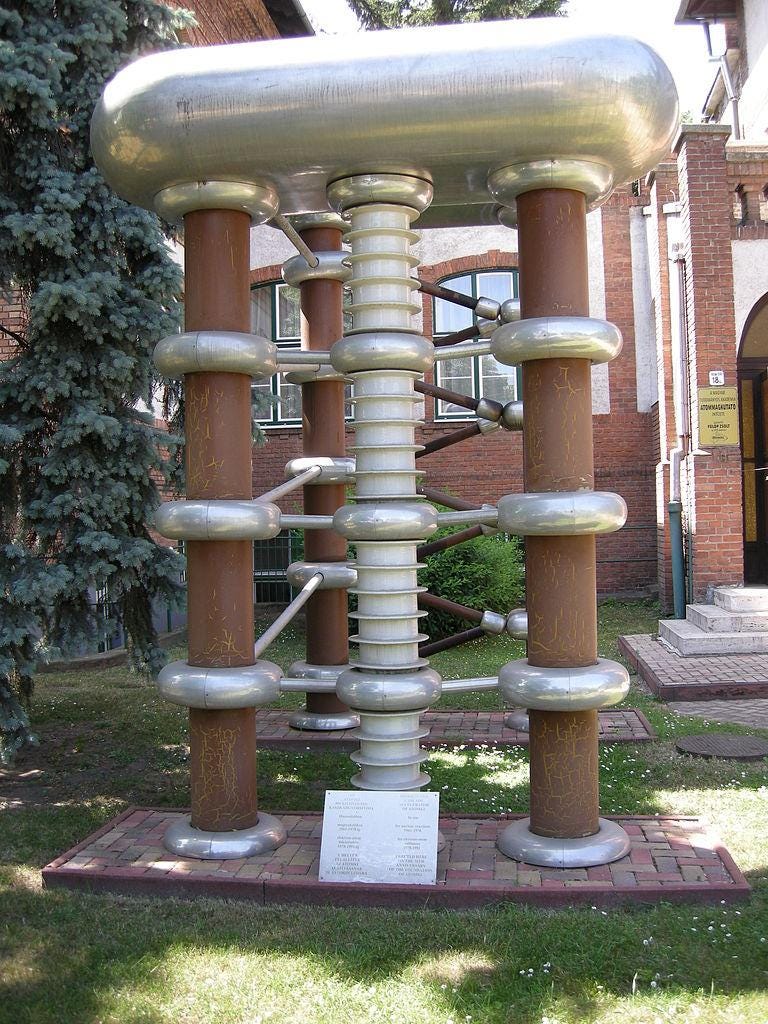
When you create a particle like beryllium-8, you fully expect it to decay back into two helium-4 nuclei with no preferred direction with respect to its center of mass. In a laboratory setting, fusing two helium-4 nuclei is impractical, but fusing lithium-7 with a proton will do just as good a job at creating beryllium-8 with one additional exception: it will create the beryllium-8 nucleus in an excited state.
Just as the Hoyle state of carbon was an excited state, it needed to emit a high-energy (gamma ray) photon before dropping down to the ground state. Well, the excited beryllium-8 has to emit a high-energy photon before it can decay to two helium-4 nuclei, and that photon will be energetic enough that there’s a chance it can spontaneously produce an electron/positron pair. The relative angle between the electron and the positron, assuming you make a detector to trace out those tracks, will tell you what the energy of the emitted photon was.
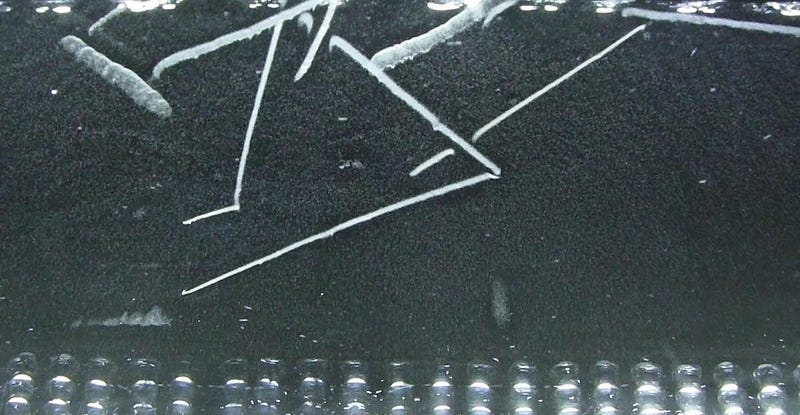
You’d fully expect that there would be a predictable energy distribution for the photon, and hence a smooth distribution in the opening angles between the electron and positron. You’d fully anticipate a maximum number of events with a particular angle, and then the event rate would decrease the greater you departed from that angle.
Except, starting in 2015, a Hungarian team led by Attila Krasznahorkay found a surprise: as the angle between the electrons and positrons gets larger, the number of events decreases, until you get to about a 140º angular separation, where they observed a surprising increase in the number of events. Maybe it was an experimental error; maybe there was an analysis error; or maybe, just maybe, the result is robust, and this is a clue that might help us solve a deep mystery in physics.
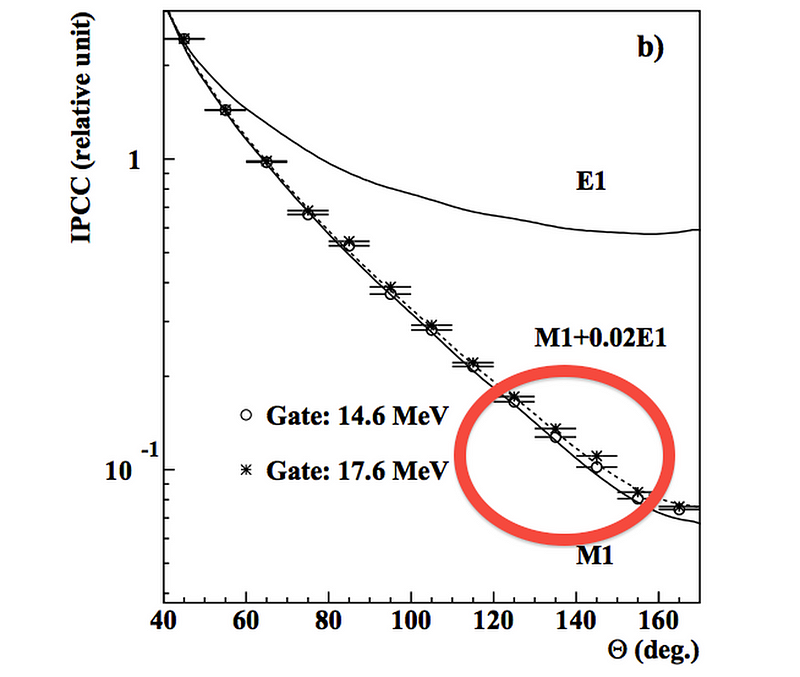
If the result is robust, one potential explanation is the existence of a new particle with a specific mass: about 0.017 GeV/c². This particle would be heavier than the electron and all of the neutrinos, but lighter than every other massive, fundamental particle ever discovered. Many differenttheoretical scenarios have been proposed to account for this measurement, and various ways to look for an experimental signature have also been devised.
When you hear about experiments looking for a dark photon, a light vector boson, a protophobic particle, or the force-carrying particle for a new, fifth force, they’re all looking for variants that could explain this Atomki anomaly. Not only that, but many of them also seek to solve one of the big puzzles with this particle: the dark matter puzzle. There’s no harm in shooting for the Moon, but every measurement has met with the same disappointment: null results.
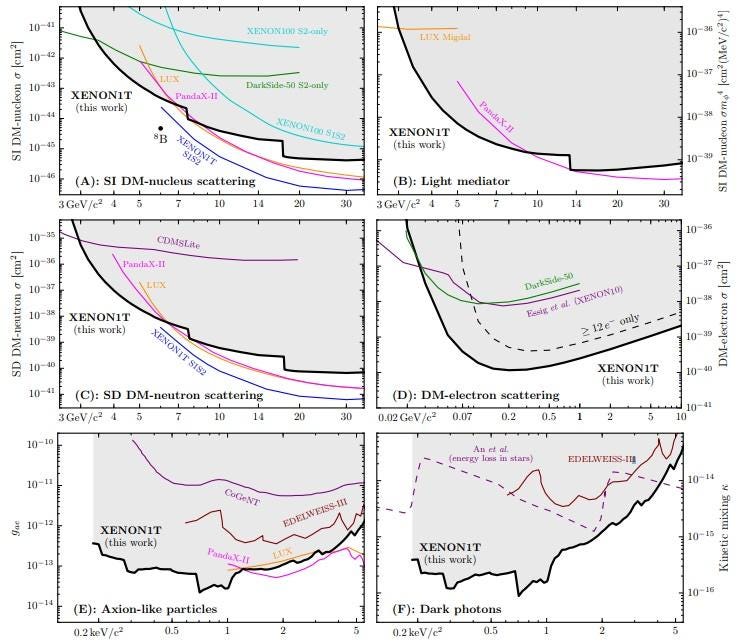
If it weren’t for the puzzling nature of the Atomki anomaly, there would be no motivation to be interested in dark matter at these energies. Results from electron-positron colliders should have seen something at these energies long ago, but no evidence for a new particle exists. It’s only through contrived scenarios, which were explicitly contrived to both explain the Atomki anomaly and evade the existing constraints, that we concocted these light dark matter scenarios.
Still, that’s where the clues are, so that’s one of the places we’re looking. There’s a big warning here: in science, we have a tendancy to find the particles we’re seeking in the places where we’re actively looking, whether they actually exist or not. Fokke de Boer, who led the Atomki experiments before Krasznahorkay did, had a rich history of discovering similar evidence for new particles, only to have those results fail verification and replication.
The jury is still out on whether this anomaly is as good as it’s hyped to be, but until we have a robust explanation, we have to both keep an open mind and look everywhere the data tells us new physics might reasonably be. Despite the null results, the search continues.
Ethan Siegel is the author of Beyond the Galaxy and Treknology. You can pre-order his third book, currently in development: the Encyclopaedia Cosmologica.