This Is Why ‘Physical Cosmology’ Was Long Overdue For The 2019 Nobel Prize

In the mid-20th century, ‘physical cosmology’ was considered an oxymoronic joke. Today, it’s Nobel-winning science.
Imagine you wanted to know everything you could about the Universe. You’d want to find the answers to questions of all types, such as:
- What is the Universe made of?
- How big is the entire Universe?
- How long has the Universe existed?
- What was the Universe like in its earliest stages?
- What types of structures exist, and when/how did they form?
- How many galaxies do we have?
- How did the Universe grow up to be the way it is today?
- And what is its ultimate fate in the far future?
It’s a daunting task to ponder. And yet, there’s a method of thinking that can give you the answer to all of these questions and many more: the method of applying physical cosmology. Earlier this October, the 2019 Nobel Prize in Physics was awarded jointly to Michel Mayor and Didier Queloz (for exoplanet discoveries) and Jim Peebles (for physical cosmology). While exoplanets are easy to understand — planets outside of our own Solar System — physical cosmology needs an explanation. Here’s the amazing story.
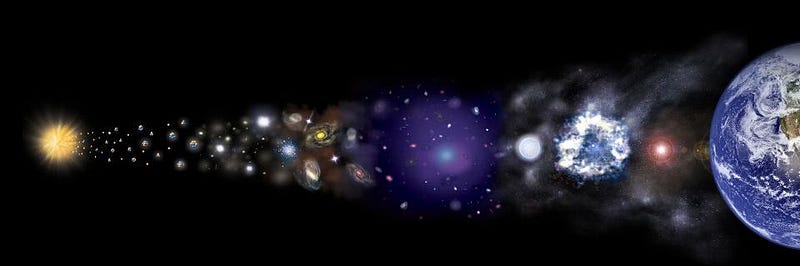
If you want to understand any object or phenomenon in the Universe, there are many different lines of approach. You can observe it in all the different ways you can imagine. This includes detecting its light in different wavelength bands; looking for spectroscopic signatures of various elements; measuring observable properties that are linked to intrinsic properties; measuring its redshift; searching for particles or gravitational waves emitted from it, etc.
However, regardless of what you’re measuring, one fact remains true about any and all structures and objects in existence: they all formed naturally in a Universe governed by the same laws and made up of the same components everywhere. Somehow, natural, physical processes occurred to take the Universe from how it was at early times and transformed it into the objects and phenomena we observe today. The key for physical cosmology is to figure out how.
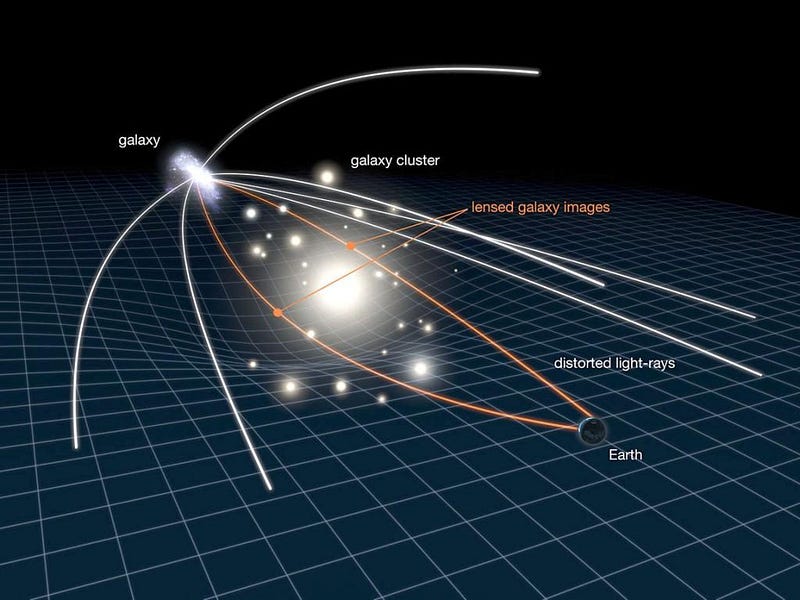
Imagine the Universe as a scientist might have imagined it a century ago: shortly after the arrival and first confirmation of General Relativity. Before any other observations are considered — and scientists were still (at the time) debating whether the Milky Way galaxy was the entirety of the Universe or whether those fuzzy spirals and ellipticals were actually their own galaxies far beyond our own (spoiler: they are) — the seeds of modern physical cosmology had already sprouted.
In physical cosmology, what you do is you start with:
- the known laws of physics,
- the relevant physical ingredients for the system you’re considering,
- the initial conditions of your physical system that your Universe begins with,
- and an accurate model for the interactions among the ingredients (including the background of spacetime).
Once you have all of that, then you do the calculations to derive what you expect to exist within our Universe.
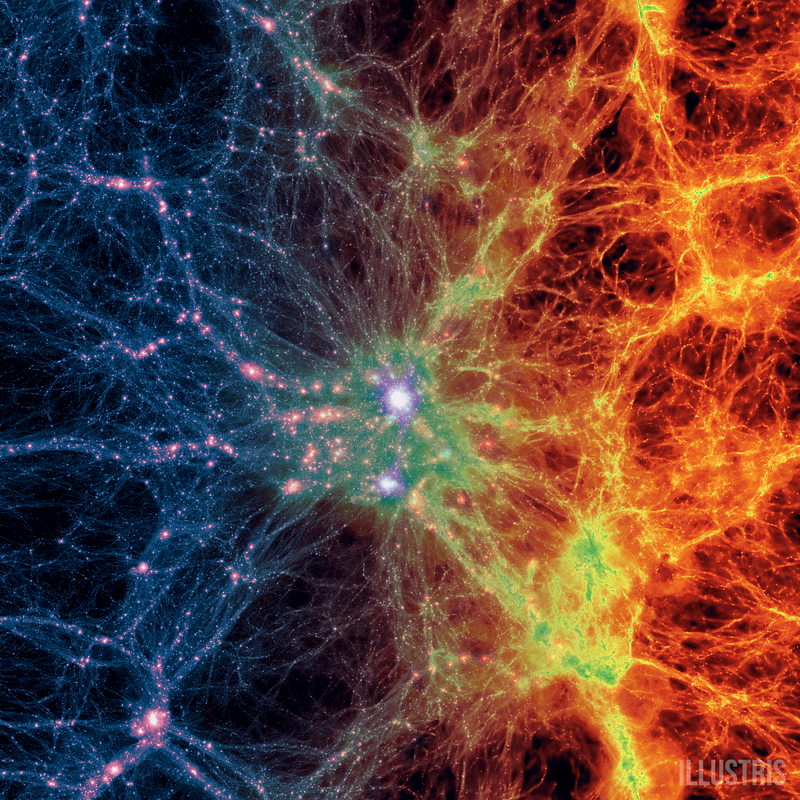
When your observations come in, you compare them with your theoretical expectations. Where observational and theoretical cosmology meet is where we, at long last, can scientifically determine what does and doesn’t accurately describe our Universe.
In the earliest days of General Relativity, the science of physical cosmology was in its most rudimentary stages. But even a primitive beginning is still a beginning, and what scientists began to derive were classes of exact solutions in General Relativity. In other word, you can make a simplifying assumption about what the properties of the Universe are, and you can write down the equations that describe a Universe that obeys those conditions under our best laws of gravity. By the end of the 1920s, we had solutions for:
- a Universe that was empty (Milne Universe),
- a Universe that contained one point mass (a non-rotating, Schwarzschild black hole),
- a Universe that contained a cosmological constant (de Sitter space),
- a Universe that was filled with normal matter alone (an Einstein-de Sitter Universe),
- and, generally, a Universe that could be filled with anything, so long as it was isotropic (the same in all directions) and homogeneous (the same at all locations in space).
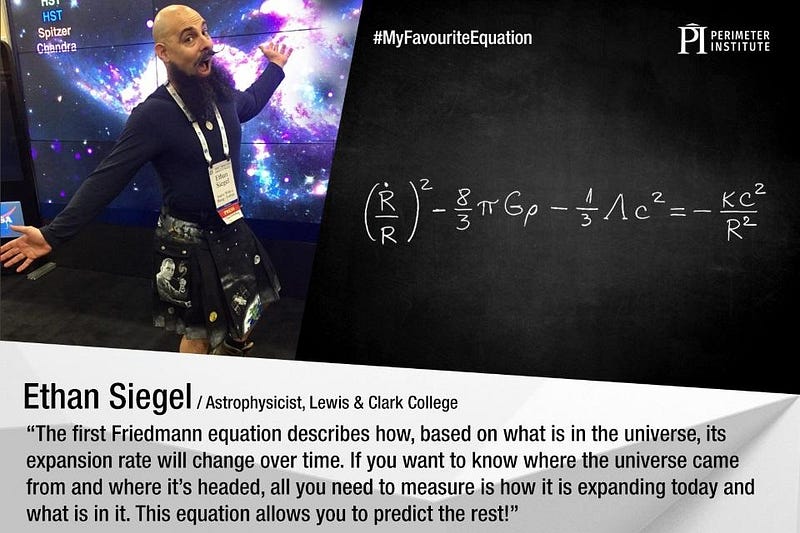
That last option gives rise to a set of equations — the Friedmann equations — with a number of fascinating consequences. First off, they predict an expanding or contracting Universe; a static one is unstable. Second, they teach you how the different possible types of energy density (e.g., normal matter, dark matter, neutrinos, radiation, dark energy, domain walls, cosmic strings, spatial curvature, and anything else you can cook up) will not only evolve with time, but they will describe how the expansion rate changes over our cosmic history as well.
By measuring not only how quickly the Universe is expanding today, but by measuring (through a variety of observational techniques) how the expansion rate has changed over time, we can begin to extract some detailed information concerning what makes up our Universe.
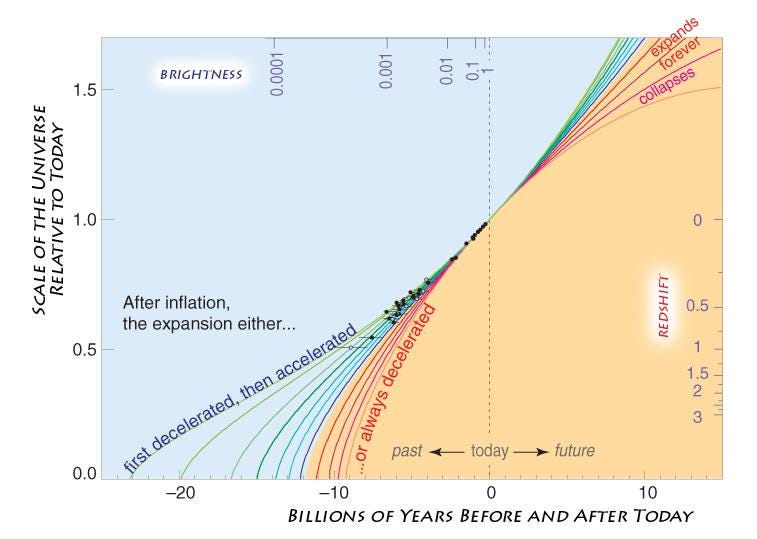
So yes, by measuring how quickly the Universe is expanding and how that expansion rate has changed over time, we can infer what the Universe’s density is, what the various components are that it’s made out of, and even — if we can determine those parameters precisely enough — what the ultimate fate of the Universe would have to be. This is the most basic example of physical cosmology: applying the laws of physics to the entirety of the expanding Universe.
Of course, this approximation is going to be good for some thing and not others. On average, it should be able to tell you how the Universe is expanding on the largest scales of all. But for all the other consequences, we have to consider some of the physical properties and particle interactions that must occur, but that we purposefully omitted earlier.
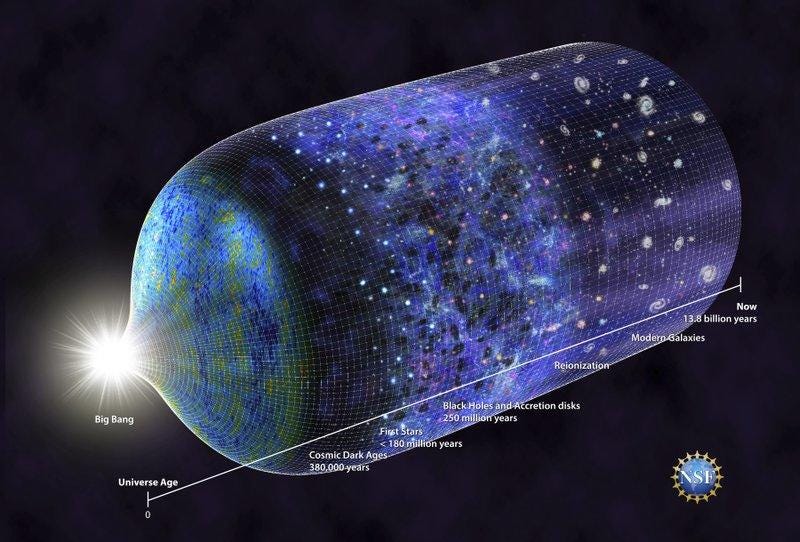
One thing we can do is to consider a Universe that includes normal matter (including protons, neutrons and electrons) and radiation (such as photons), as well as the interactions that govern such particles. When the Universe started off, it was mostly uniform, but also contained this matter and this radiation. It was also hotter, as an expanding Universe stretches the wavelengths of photons within it, making them less energetic over time.
If we extrapolate back to the past, we can calculate that there was an early time (and a high enough temperature) in the Universe’s past where the formation of neutral atoms would have been impossible, as photons would have blasted them apart into an ionized state. In order to calculate when that occurred, you have to calculate all the atomic physics necessary to learn when the Universe’s atoms become stably neutral, and how that impacts what we’ll see today as the leftover radiation from the Big Bang: the cosmic microwave background (CMB).
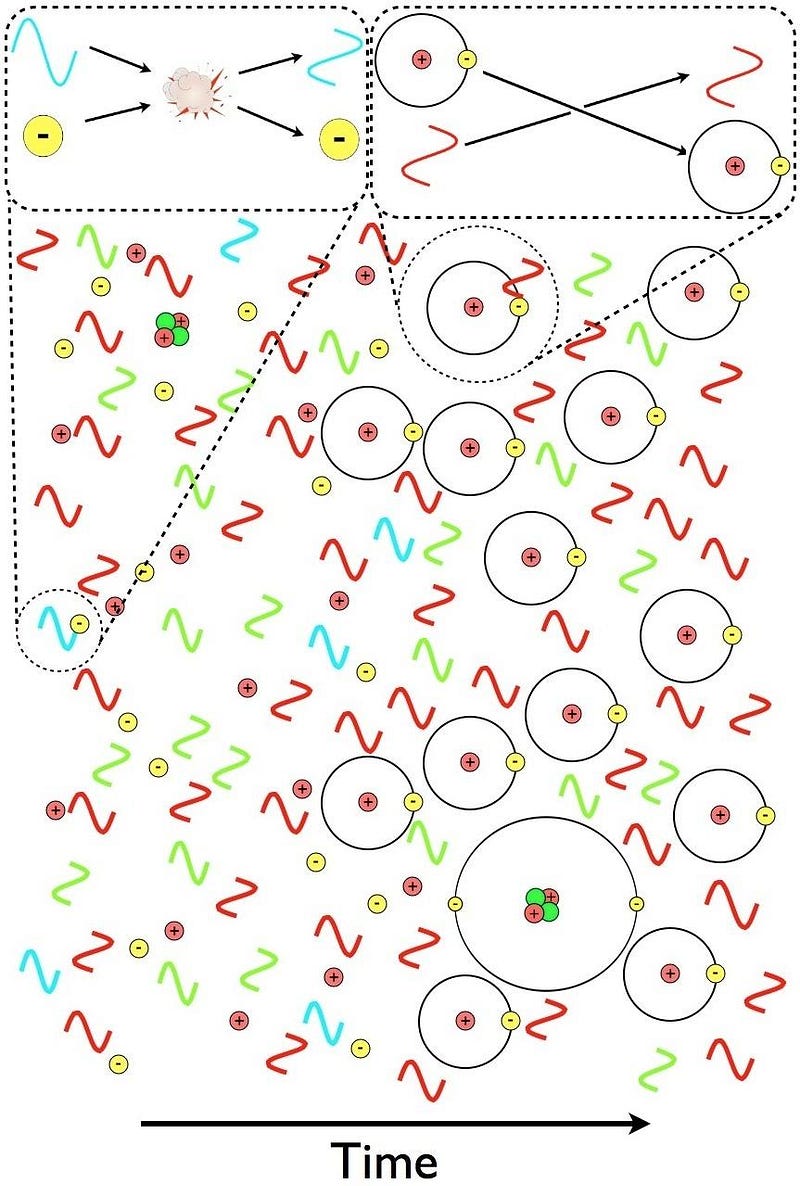
At even earlier times, you can perform the analogous calculation for atomic nuclei, and see where collisions blast composite nuclei apart into protons and neutrons versus where they’re no longer energetic enough to do so. When you then go and measure the abundances of these light elements (by probing gas clouds that have never formed stars), you should see a specific ratio of elements like hydrogen, deuterium, helium-3, helium-4, and lithium-7.
If you go even earlier and understand that the early Universe must have had high enough energies to spontaneously produce matter-antimatter pairs (and understand how fermions like neutrinos obey different rules than bosons like photons), you can calculate the ratio of the cosmic neutrino background’s energy to the CMB’s individual photon energy, since when the electron-positron pairs from the early Universe annihilate away, they only become photons, never neutrinos. The calculation tells us the neutrino temperature is (4/11)⅓ times the CMB temperature; since the latter is 2.725 K, the former must have a temperature equivalent of 1.95 K.

Physical cosmology also tells you what sort of structures you expect to find in the Universe. You can start with an almost-homogeneous Universe, but one that has density (and/or temperature) imperfections in it, model the interactions between particles and radiation and include gravitation, and see how these imperfections evolve.
You’ll find that the imperfections evolve according to different behaviors depending on how much normal matter vs. dark matter exists in your Universe, and will leave a specific imprint in the CMB. You’ll find that the overdense regions grow at a quantifiable rate until they reach a critical density, then undergo runaway collapse to form stars, galaxies, and other cosmic structure. The early stars reionize the Universe; the larger-scale structure forms today’s massive cosmic web.
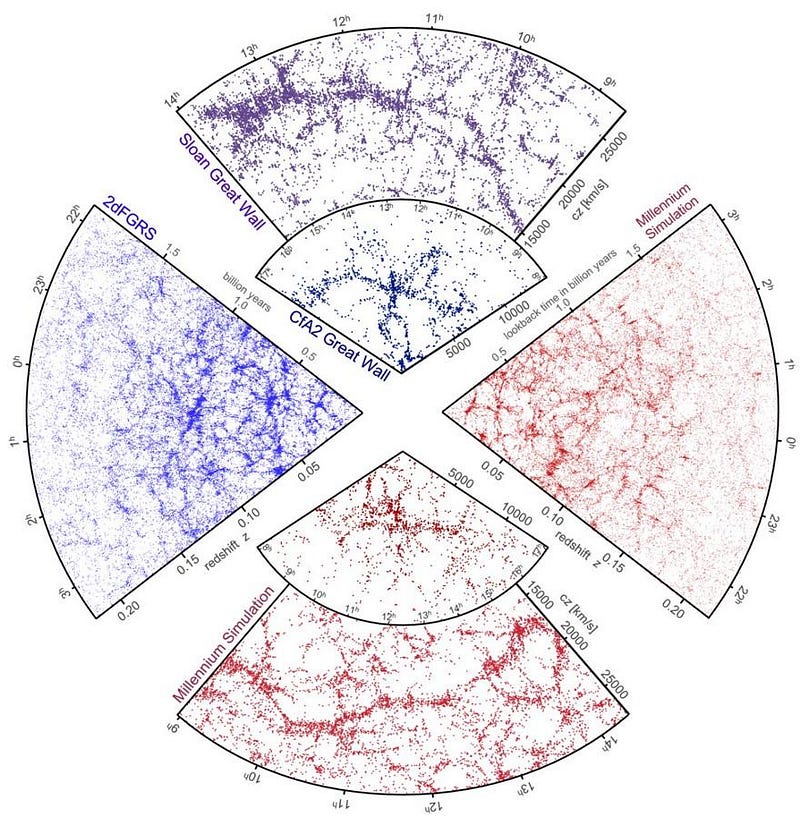
It is a spectacular fact of modern science that the predictions of theoretical cosmology have been verified and validated by ever-improving observations and measurements. Even more remarkably, when we examine the full suite of the cosmic data humanity has ever collected, one single picture accurately describes every observation together: a 13.8 billion year old Universe that began with the end of cosmic inflation, resulting in a Big Bang, where the Universe is comprised of 68% dark energy, 27% dark matter, 4.9% normal matter, 0.1% neutrinos, and a tiny bit of radiation with no spatial curvature at all.
Put those ingredients into your theoretical Universe with the right laws of physics and enough computational power, and you’ll obtain the vast, rich, expanding and evolving Universe we have today. What was initially an endeavor of just a handful of people has now become the modern precision science of cosmology. In the middle of the 20th century, legendary physics curmudgeon Lev Landau famously said, “Cosmologists are often in error but seldom in doubt.” With the 2019 Nobel Prize in Physics going to Jim Peebles, perhaps the world will recognize it’s long past time to retire Landau’s quote. We may live in a dark Universe, but the science of physical cosmology has shed a light on it like nothing else.
Ethan Siegel is the author of Beyond the Galaxy and Treknology. You can pre-order his third book, currently in development: the Encyclopaedia Cosmologica.