This Black Hole Paradox Should Prohibit The Most Massive Ones From Existing
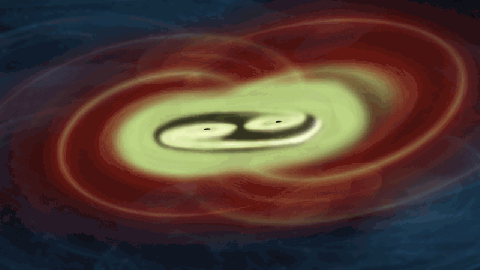
The ‘final parsec problem’ is still a mystery for astronomers.
When it comes to black holes in the Universe, we know there are at least two major types. There are the low-mass black holes, which arise from the deaths of individual, massive stars or the mergers of two stellar remnants, like neutron stars. There are also the supermassive black holes found at the centers of galaxies, where practically every large, massive galaxy seems to have one.
Ever since the advanced LIGO detectors opened their gravitational “eyes” on the Universe in 2015, we’ve witnessed a plethora of black hole-black hole mergers, all of the low-mass type. With just a few years of observations behind us, we’ve already observed more than 60 such mergers, confirming numerous predictions of Einstein’s relativity to spectacular precision.
However, the same gravitational physics that predicts the mergers of these low-mass black holes predicts that, when two galaxies — each with supermassive black holes — merge together, their black holes will stall out and won’t merge. For the past few years, astronomers have called this the final parsec problem, and it’s one of the most contentious, but unsung, paradoxes in all of physics. Here’s what’s at stake.
When we see two black holes merge, what is it that’s going on?
For most of us, our first instinct is to imagine each and every galaxy as being filled with stars, each one carving their own unique orbital path through the galaxy. The hottest, bluest, most massive stars burn through their fuel the fastest, dying the most quickly and winding up as either a neutron star or a black hole: the end result of a type II supernova explosion.
You can easily imagine that, in the gravitational dance of each galaxy, occasionally two of these stellar remnants will someday collide with one another, leading to either a:
- neutron star-neutron star,
- neutron star-black hole, or
- black hole-black hole
merger. This is a totally reasonable line of thought, and actually is a process that’s thought to occur. However, the percentage of stellar remnants that merge this way is so thoroughly rare that it’s completely negligible. When we look at the mergers that we’ve directly observed, in fact, it appears that zero of them have merged in this fashion; another pathway completely dominates.
Of all the stars that we’ve observed in the Universe, only approximately half of them are in systems like our Sun: where a single, central star is orbited by planets and other objects. The other half reside in multi-star systems, like binaries or trinaries, or in a small percentage of cases, even greater numbers of stars. Although many of the systems we’ve observed contain stars of very different masses, a large fraction of these systems consist of stars of similar masses. Since mass is the primary arbiter of a star’s fate, that means if one member of a binary (or greater) system becomes a black hole or neutron star, another member is quite likely to do so as well.
Whenever you have two black holes — or, for that matter, any two masses — orbiting one another, something subtle but profound occurs: their orbits will decay. Every time one mass moves through a changing gravitational field, a tiny amount of energy gets emitted in the form of gravitational radiation, and that carried-away energy causes that mass to lose a little bit of its energy. Over long enough timescales, all gravitationally bound orbits will decay away, causing any two masses to spiral into one another.
For well-separated masses that are relatively small, like the Sun and the Earth, it will take much, much longer than the age of the Universe for such a process to occur. Although it’s been a substantial amount of time since the Big Bang — 13.8 billion years, to be precise — it will take the Earth somewhere around ~10²⁶ years for its orbit to decay via gravitational radiation and spiral into the Sun. For larger mass systems, though, and/or for systems with smaller separation distances, this timescale gets reduced dramatically.
Many of the stars that we observe in the Universe have rather tight orbits, including a substantial fraction of the rare, high-mass binary systems we see. If we extrapolate these systems into the future, we fully expect that there will be a substantial fraction of them born close enough together that they can explain the currently observed rates of:
- neutron star-neutron star mergers,
- black hole-neutron star mergers,
- and black hole-black hole mergers,
at least for the types of black holes that LIGO (and other terrestrial gravitational wave observatories) are sensitive to.
When we scale this up to larger black holes, we find that the same type of physics applies. When you have a significantly large mass moving through the (changing) gravitational field generated by another mass, it will emit gravitational radiation, carrying energy away and causing the orbits to decay. The greater your masses and the smaller the separation distance between them, the greater the rate of this orbital decay is predicted to be. While there are numerous examples of stellar mass black holes — black holes of ~100 solar masses or less — meeting the right conditions for this orbital decay to lead to inspirals and mergers, the situation is a lot murkier for the behemoths at the centers of galaxies: populated by supermassive black holes.
Lurking in the central cores of galaxies, supermassive black holes range from a few million up to tens of billions of solar masses, with the size of the black hole’s event horizon (and the rate of gravitational radiation) increasing with mass. For the largest, most massive black holes of all, their event horizons are comparable in scale to our entire Solar System. If we ask the question, “how well-separated can two supermassive black holes be and still inspiral and merge in less than the age of the Universe?” the answer we obtain is somewhere around ~0.01 light-years, or a few thousand times the current distance separating the Earth and the Sun.
But is this likely to occur? Can we get two supermassive black holes to be in a very tight orbit like this with one another?
The science is pretty dubious here, and it’s pretty easy to see why if we take an in-depth look at what brings two supermassive black holes together. Each galaxy, as it goes through its life cycle, develops and grows a supermassive black hole within it. It’s thought that this occurs as:
- the most massive stars form, live, and die,
- leading to seed black holes,
- which interact with the other masses within the galaxy,
- causing the lightest masses to get ejected and the heaviest masses to sink towards the center,
- where they interact, accrete, grow, and merge together,
leading to the central supermassive black holes we see today.
Then, over time, the individual galaxies gravitationally attract one another, form gravitationally bound groups and clusters of galaxies, and eventually collide and merge. When they do, they very rarely collide in a center-on-center fashion, meaning that the two black holes will miss one another. Typically, these galaxy collisions occur with enormous separation distances between the black holes, ranging from tens to tens-of-thousands of light-years.
However, a very similar process that created and grew these supermassive black holes in the first place then occurs for the masses within the newly-merged galaxy: violent relaxation. When two galaxies merge, you now have two supermassive black holes in an environment rich in matter, and in particular, rich in matter occupying the space between them. This matter includes:
- gas,
- dust,
- stars,
- stellar remnants,
- ionized plasma,
- and dark matter,
all of which is gravitationally bound to the new, larger, post-merger galaxy.
As these black holes move through the galaxy, they gravitationally interact with everything around them. Although it’s a pretty famous result that whenever you have three masses gravitationally bound together, that’s not a problem that’s exactly solvable under our theory of gravity — known as the three-body problem — we still know what’s going to typically happen. If you have two large masses (like two supermassive black holes) interacting with a third, smaller mass (like, literally anything else in between them in a galaxy), the smaller mass gets kicked out, bringing the two larger masses closer together and into a more tightly bound orbit.
Both violent relaxation and dynamical friction will eject copious amounts of matter and draw the two black holes in a post-merger galaxy close together. But if we want to know what happens, there’s a problem. We can’t sit here from our perspective within the Milky Way and simply watch galaxies evolve over these cosmically long timescales, however; time passes elsewhere in the Universe at the same rate it passes for us. Therefore, if we want to know what happens to these black holes as they orbit one another, we have to resort to simulations, identifying what happens as these various masses interact over timescales far in excess of what we’re able to observe.
What we generally find is that whenever we have two galaxies, each with their own supermassive black holes, and they collide and merge, the following steps occur.
- The black holes begin moving with very high speeds, high enough that they’re in danger of getting ejected.
- However, dynamical friction, which is the gravitational “braking” that occurs from large masses plowing through gas, dust, and plasma, slows them down.
- Additional gravitational interactions cause these black holes to sink towards the center, losing kinetic energy and ejecting or kicking to higher orbits the matter they interact with.
- And finally, they enter an orbital state where they’ve ejected all of the matter interior to their mutual orbit.
The major problem with this scenario? The black holes don’t get close enough to inspiral-and-merge in less than the age of the Universe.
The processes that we know of can pretty much always get black holes to within a few parsecs of one another, where one parsec is ~3.26 light-years. In the best case scenarios, these two black holes can get quite close, to within about ~0.1 light-years of one another, while they’re almost never left more than about ~10 light-years apart. Still, that’s very far off from the ~0.01 light-years or less that these black holes require in order to inspiral and merge within the age of the Universe.
And yet, when we look at the black holes we see in the centers of galaxies, we don’t see any evidence that they come in binary pairs. Instead, we see things consistent with one large behemoth, like what we’ve observed at our own galaxy’s core or — quite directly with the Event Horizon Telescope — the center of the giant nearby elliptical galaxy, M87.
There are plenty of possibilities for how they could get there. Perhaps whenever we have two galaxies merge, there are usually others that come along as well, and the introduction of a third (or more) supermassive black hole enables the two largest to get close enough to merge. Perhaps gas, dust, or stars sink to the galaxy’s center too, where over time, they draw the black holes close together enough that they’ll merge. Or, quite possibly, perhaps in most cases, the two black holes don’t actually merge, but continue to orbit one another below the limit at which our telescopes can resolve them. With the next generation of telescopes slated to come online in the coming decades, we might actually find if these “tight, but not tight enough, black hole binaries” are the norm, rather than the exception.
Still, it’s worth emphasizing that when we examine the supermassive black holes at the centers of galaxies in detail, which we can do most effectively for nearby and active galaxies, they appear to be dominated by just one black hole. Observationally, that’s what we conclude is present. And yet, we think we know what galaxies are made of, how gravitation works, and how to simulate the interactions between black holes and other massive forms of matter. Our theoretical predictions indicate that when galaxies merge, their black holes should come within 0.1-to-10 light-years of one another, but no closer. That’s not close enough to inspiral and merge from the emission of gravitational waves, leading to a paradox: the final parsec problem.
So how, then, does the Universe manage to create the supermassive black holes that we see? Perhaps we’re underestimating the effects of the accretion of matter from intergalactic space, or the funneling of matter into the inner reaches of galaxies. Perhaps multiple mergers are more common than we realize, and that there are many more large black holes at play than just two. Or — and this is tantalizing — it’s possible that there are many binary supermassive black holes out there, just not quite resolvable with current technology.
Only time, superior observations, and better science will teach us what the solution is. In the meantime, hold all the possibilities together in your head when you think about the puzzle, and marvel that, at least in some cases, the Universe does find a way to overcome this paradox!
Starts With A Bang is written by Ethan Siegel, Ph.D., author of Beyond The Galaxy, and Treknology: The Science of Star Trek from Tricorders to Warp Drive.