These 4 Pieces Of Evidence Have Already Taken Us Beyond The Big Bang
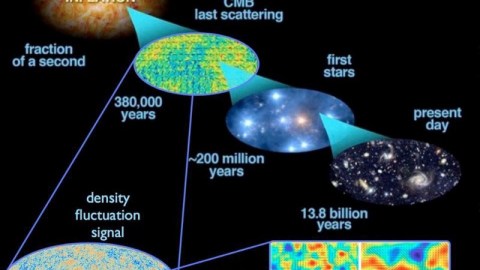
Sure, cosmic inflation has its detractors. But it also has something no alternative possesses: predictions and tests.
Perhaps the most compelling part of any remarkable story is its origin: how it all began. We can take that question back as far as we want, asking what came before and gave rise to whatever we were asking about previously, until we find ourselves pondering the origin of the Universe itself. This is perhaps the greatest origin story of all, which occupied the minds of poets, philosophers, theologians and scientists for countless millennia.
It was only in the 20th century that science began to make progress on that question, however, eventually resulting in the scientific theory of the Big Bang. Early on, the Universe was extremely hot and dense, and has expanded, cooled, and gravitated to become what it is today. But the Big Bang itself wasn’t the beginning, after all, and we have four independent pieces of scientific evidence that show us what came before it and set it up.
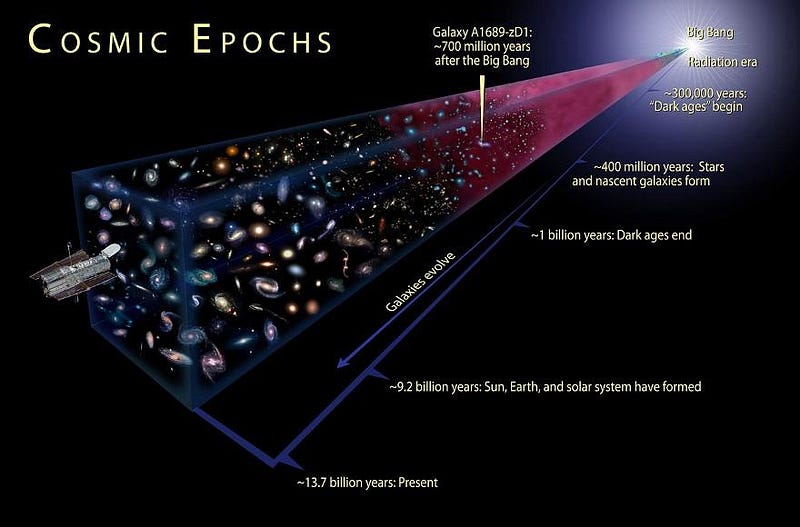
The Big Bang was an idea that was first loosely conceived way back in the 1920s, in the early days of General Relativity. In 1922, Alexander Friedmann was the first to recognize that if you had a Universe that was uniformly filled with matter-and-energy all throughout it, with no preferred directions or locations, it couldn’t be static and stable. The fabric of space itself, under Einstein’s laws, had to either be expanding or contracting.
In 1923, Edwin Hubble made the first distance measurement of Andromeda, demonstrating for the first time that it was a galaxy completely outside of the Milky Way. By combining his measurement of galactic distances with the redshift data of Vesto Slipher, he could actually measure the Universe’s expansion directly. In 1927, Georges Lemaître became the first to put all the pieces together: an expanding Universe today implied a smaller, denser past, going as far back as we dared to extrapolate.
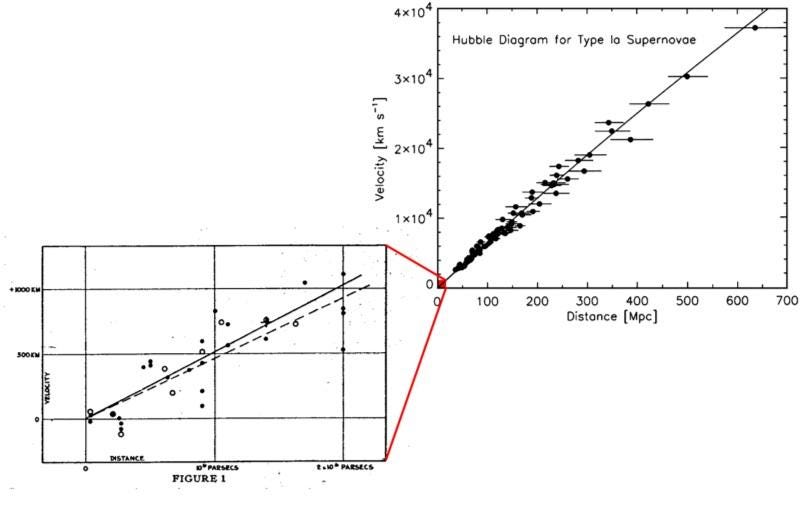
Beginning in the 1940s, George Gamow and his collaborators started working out the consequences of a Universe that was expanding and cooling today, but hotter and denser in the past. In particular, he derived four major results.
- The Universe’s expansion rate would evolve over time, dependent on what types and ratios of matter-and-energy were present.
- The Universe would have undergone gravitational growth, where initially small overdensities would, in time, grow into stars, galaxies, and the great cosmic web.
- The Universe, being hotter in the past, would have at some early time been hot enough to prevent neutral atoms from forming, meaning that there should be a leftover glow of radiation emitted when those neutral atoms finally formed.
- And, even earlier on, it should have been hot and dense enough to ignite nuclear fusion between protons and neutrons, which should have created the first non-trivial elements in the Universe.
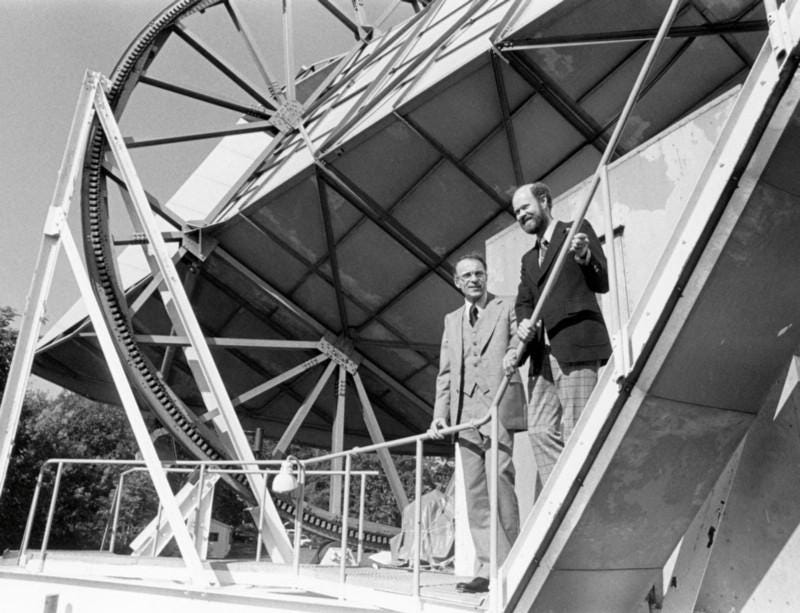
In 1964 and 1965, two radio astronomers at Bell Labs, Arno Penzias and Robert Wilson, discovered a faint glow of radiation emanating from all directions in the sky. After a short period of surprise, confusion, and mystery, this signal was found to match the prediction of radiation from the Big Bang. Subsequent observations over the coming decades revealed even more precise details, matching the Big Bang’s predictions to great accuracy.
The growth and evolution of galaxies and large-scale structure in the Universe, measurements of the expansion rate and temperature changes over the Universe’s evolutionary history, and the measurement of the abundances of the light elements all matched within the framework of the Big Bang. By every metric where data existed, the Big Bang was a smashing success. Even today, no alternative theory has reproduced all of these successes.
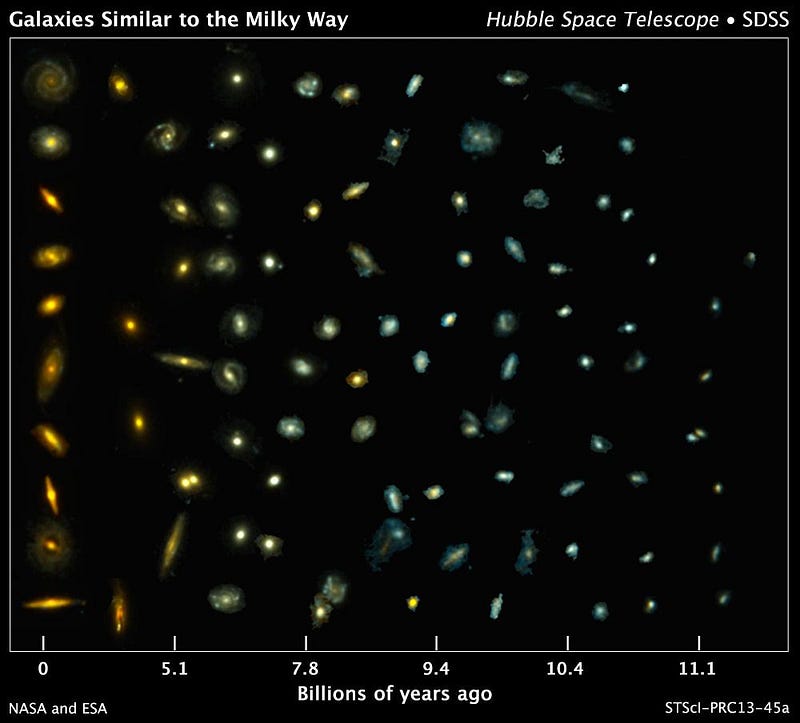
But how far back can you take the idea of the Big Bang? If the Universe is expanding and cooling today, it must have been hotter, denser, and smaller in the past. The natural instinct is to go back as far as the laws of physics — like General Relativity — will allow you to go: all the way back to a singularity. At one particular moment, the entirety of the Universe would be compressed into a single point of infinite energy, density, and temperature.
This would correspond to the idea of a singularity, which is where the laws of physics break down. It’s conceivable that this is where space and time were first created. And, owing to our modern understanding of our Universe, we can extrapolate all the way back to one particular moment a finite amount of time ago: 13.8 billion years. If the Big Bang were all that there was, this would be the ultimate origin of our Universe: a day without a yesterday.
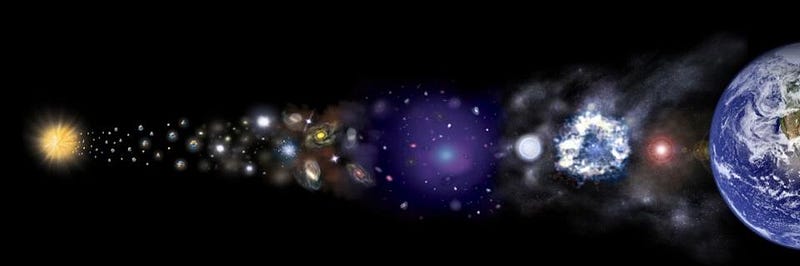
But the Universe as we see it has some properties — and some puzzles — that the Big Bang doesn’t explain. If everything began from a singular point a finite amount of time ago, you’d expect:
- different regions of space would have different temperatures, since they wouldn’t have had the ability to communicate and exchange particles, radiation, and other forms of information,
- leftover particle relics from the earliest, hottest times, such as magnetic monopoles and other topological defects,
- and some degree of spatial curvature, since a Big Bang that arises from a singularity has no way to balance the initial expansion rate and the total matter-and-energy density so perfectly.
But none of these things are true. The Universe has the same temperature properties everywhere, no leftover high-energy relics, and is perfectly spatially flat in all directions.
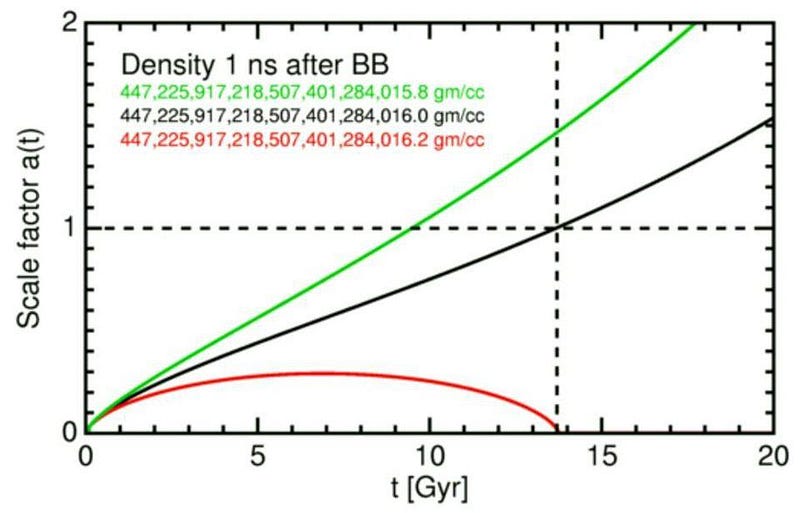
Either the Universe was simply born with these properties for no foreseeable reason at all, or there is a scientific explanation: a mechanism that caused the Universe to come into existence with these properties already in place. On December 7, 1979, physicist Alan Guth had a spectacular realization: an early period of exponential expansion that preceded the Big Bang — what we now know as cosmic inflation — could have caused the Universe to be born with all of these specific properties. When inflation came to an end, that transition should then give rise to the hot Big Bang.
Of course, you can’t just build an extra idea into your old theory and declare that your new one is better. In science, the burden of proof on the new theory is much more severe.
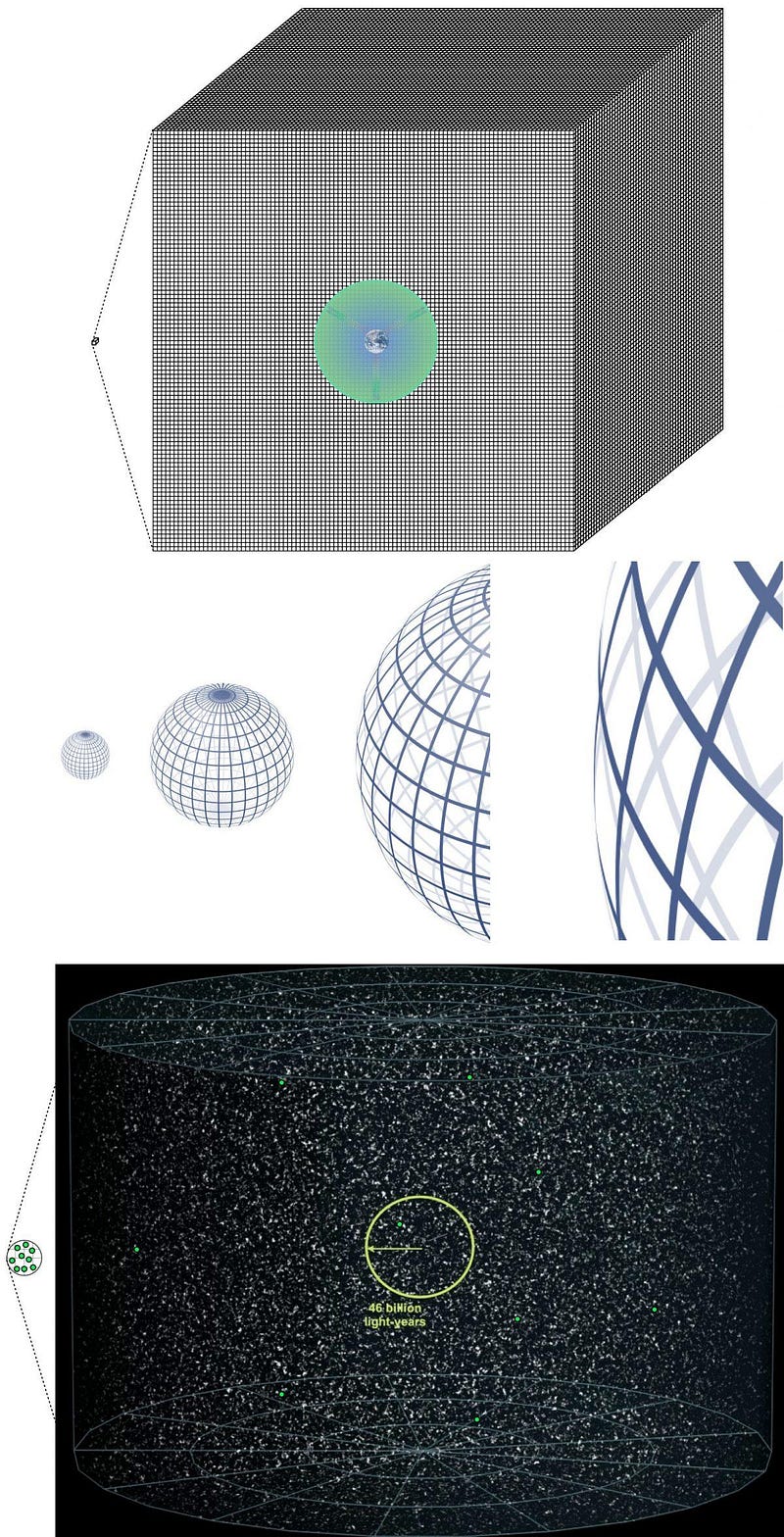
To supersede any prevailing scientific theory, a new one has to do three things:
- reproduce all of the pre-existing theory’s successes,
- explain the mysteries that the old theory could not,
- and make new, testable predictions that differ from the previous theory’s predictions.
Over the course of the 1980s, it was clear that inflation could easily accomplish the first two. The ultimate tests would come when our observational and measurement capabilities allowed us to compare what the Universe gives us with inflation’s novel predictions. If inflation is true, we would have to not only tease out what those potentially observable consequences would be — and there are a few — but to gather that data and draw conclusions based on it.
So far, four of those predictions have been put on trial, with the data now being good enough to fully evaluate the results.
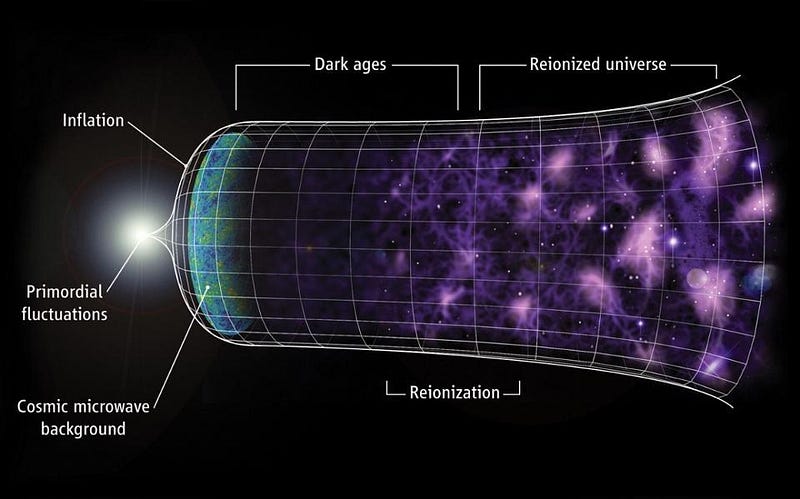
1.) The Universe should have a maximum, non-infinite upper limit to the temperatures reached in the hot Big Bang. The leftover glow from the Big Bang — the cosmic microwave background — has some regions that are slightly hotter and some that are slightly colder than average. The differences are minuscule, about 1 part in 30,000, but encode an enormous amount of information about the young, early Universe.
If the Universe underwent inflation, there should be a maximum temperature that’s equivalent to significantly lower energies than the Planck scale (~10¹⁹ GeV), which is what we’d reach in an arbitrarily hot, dense past. Our observations of these fluctuations teach us that the Universe got no hotter than about 0.1% (~10¹⁶ GeV) of that maximum at any point, a confirmation of inflation and an explanation for why there are no magnetic monopoles or topological defects in our Universe.
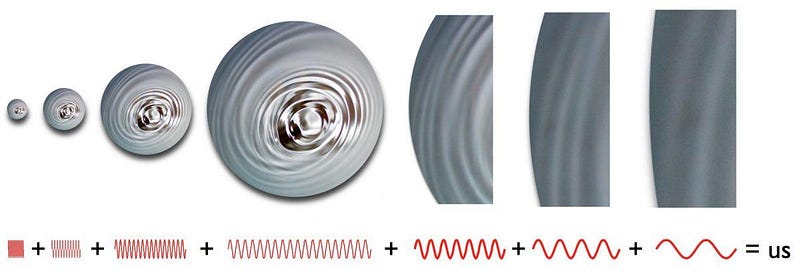
2.) Inflation should possess quantum fluctuations that become density imperfections in the Universe that are 100% adiabatic. If you have a Universe where one region is denser (and colder) or less dense (and hotter) than average, those fluctuations can either be adiabatic or isocurvature in nature. Adiabatic means “constant entropy” while isocurvature means “constant spatial curvature,” where the biggest difference is how that energy is distributed between different types of particles like normal matter, dark matter, neutrinos, etc.
This signature appears in the Universe’s large-scale structure today, allowing us to measure what fraction is adiabatic and what fraction is isocurvature. When we make our observations, we find that these early fluctuations are at least 98.7% adiabatic (consistent with 100%) and no more than 1.3% (consistent with 0%) isocurvature. Without inflation, the Big Bang makes no such predictions at all.
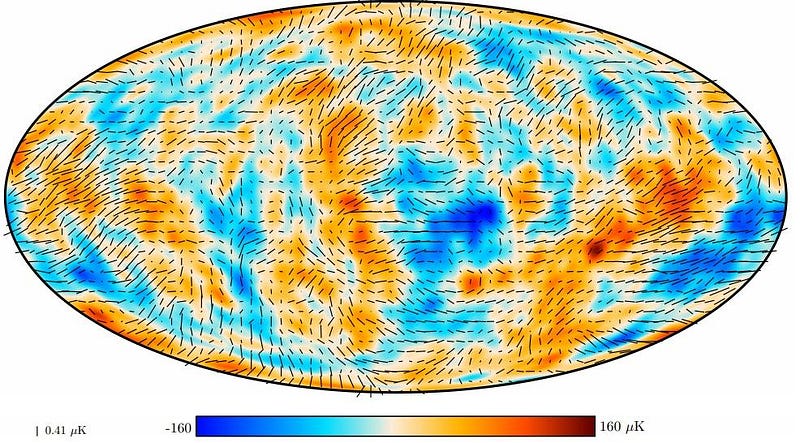
3.) Some fluctuations should be on super-horizon scales: fluctuations on scales larger than light could have traveled since the hot Big Bang. From the moment of the hot Big Bang, particles travel through space at a finite speed: no faster than the speed of light. There’s a specific scale — what we call the cosmic horizon — that represents the maximum distance a light signal could have traveled since the hot Big Bang.
Without inflation, fluctuations would be limited to the scale of the cosmic horizon. With inflation, since it stretches the quantum fluctuations that occur during this exponentially expanding phase, you can have super-horizon fluctuations: on scales larger than the cosmic horizon. These fluctuations have been seen in the polarization data provided by the WMAP and Planck satellites, in perfect agreement with inflation and running contrary to a non-inflationary Big Bang.
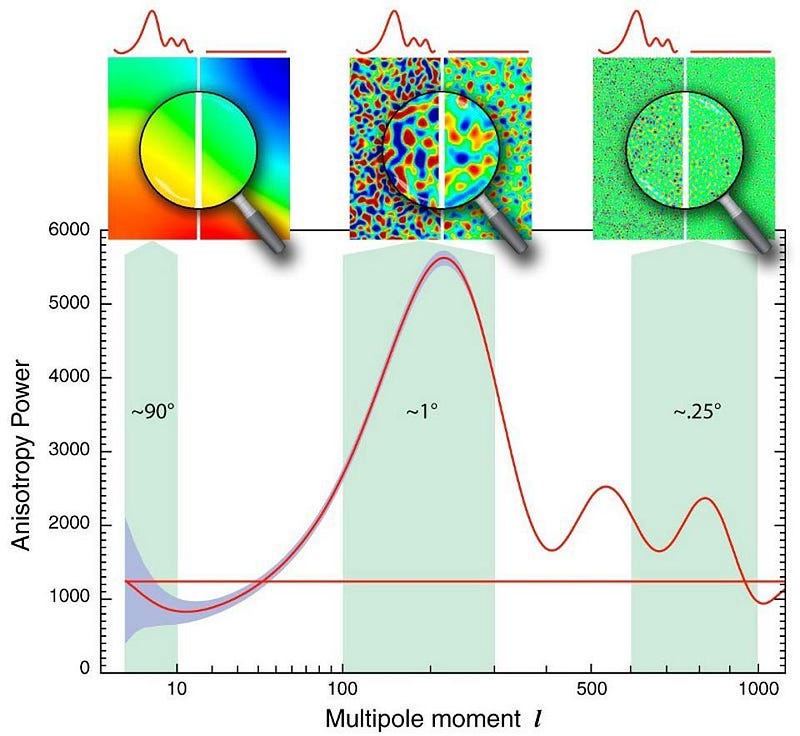
4.) Those fluctuations should be almost, but not perfectly, scale-invariant, with slightly greater magnitudes on large scales than small ones. All fundamental fields in the Universe are thought to be quantum in nature, and the field responsible for inflation is no exception. Quantum fields all fluctuate, and during inflation, these fluctuations get stretched across the Universe, where they provide the seeds of our modern cosmic structure.
In inflation, these fluctuations should be almost scale-invariant, which means they’re the same magnitude on all scales, large and small. But they should be slightly greater in magnitude, by just a few percent, on larger scales. We use a parameter called the scalar spectral index (n_s) to measure it, with n_s = 1 corresponding to perfect scale invariance. We’ve now measured it precisely: 0.965, with an uncertainty of ~1%. This slight departure from scale invariance has no explanation without inflation, but inflation predicts it perfectly.
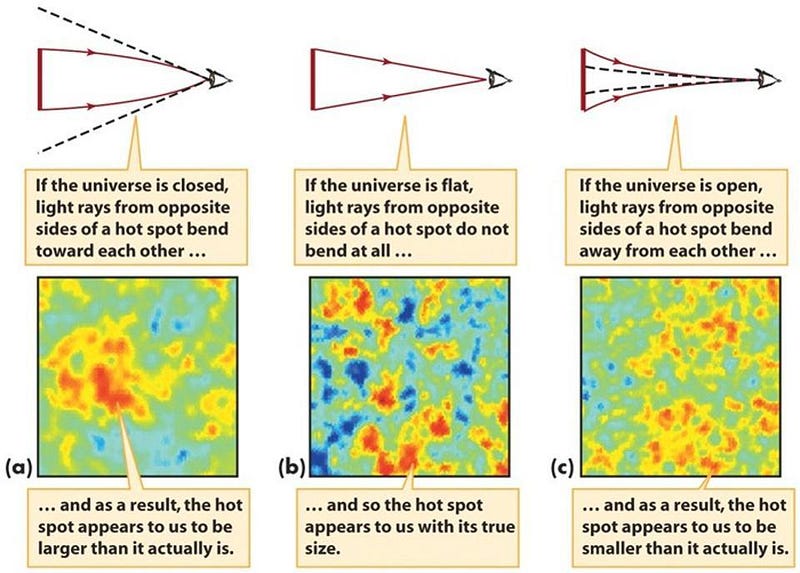
There are other predictions of cosmic inflation, too. Inflation predicts that the Universe should be almost perfectly flat, but not quite, with the degree of curvature falling somewhere within 0.0001% and 0.01%. The scalar spectral index, measured to depart slightly from scale invariance, should “roll” (or change during the final stages of inflation) by about 0.1%. And there should be a set of not just density fluctuations, but gravitational wave fluctuations that arise from inflation. So far, observations are consistent with all of these, but we have not reached the level of precision necessary to test them.
But four independent tests are more than enough to draw a conclusion. Despite the voices of a few detractors who refuse to accept this evidence, we can now confidently state that we’ve gone before the Big Bang, and cosmic inflation led to the birth of our Universe. The next question, of what happened prior to the end of inflation, is now at the frontier of 21st century cosmology.
Ethan Siegel is the author of Beyond the Galaxy and Treknology. You can pre-order his third book, currently in development: the Encyclopaedia Cosmologica.