The Temperature of Dark Matter
If we wanted to know how cold it is now and was in the distant past, how would we figure it out?
“Science casts a long black shadow back over who we think we are, and where it falls the temperature falls with it. Its touch is chilly and unforgiving.” –Richard K. Morgan
Do you remember the moment it clicked for you, when you realized there was a much finer level of detail to the world — that it was made up of something far more intricate — than you could perceive? I was maybe six or seven, and had read a book that told me that everything was made up of tiny particle called molecules, so small that you couldn’t even see them with a microscope.
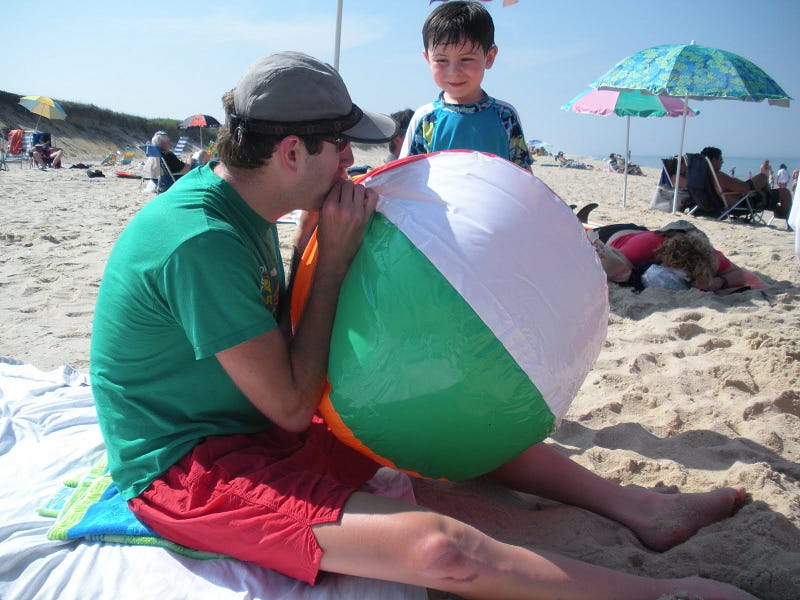
Not only were they always in motion, but that even if you couldn’t see it, they moved faster and with more energy as you heated them up. The example they gave was that when you inflated a beach ball before you went out to the beach, you should leave a little bit of room for more air inside, and the Sun would heat up the air inside, and that would inflate it the rest of the way. And it did, and when it got cool again in the evening, the beach ball deflated again a little bit.
So that was consistent with things being made of molecules and with the temperature being related to the speed of the molecules, but I wanted something more direct. A little while later, I read about a different experiment that I simply had to try: to take a glass of ice cold water and a glass of scalding hot water, and to drop a drop of food coloring in each one. If the water was made of molecules that were always moving around, and the hotter molecules were moving faster, then the food coloring should disperse much more rapidly through the hot water than the cold.
https://www.schooltube.com/video/56bf0d480ca8450e92f2/Food coloring in hot and cold water
And that’s exactly what happened! Even though I didn’t have a thermometer, and couldn’t directly measure the temperature, I recognized that it was possible to learn about the temperature of the air in the beach ball or the water in the glass by simply making the right observations.
Well, it’s a little bit less familiar, but you might ask the same question about the most mysterious and elusive substance in the Universe: dark matter!
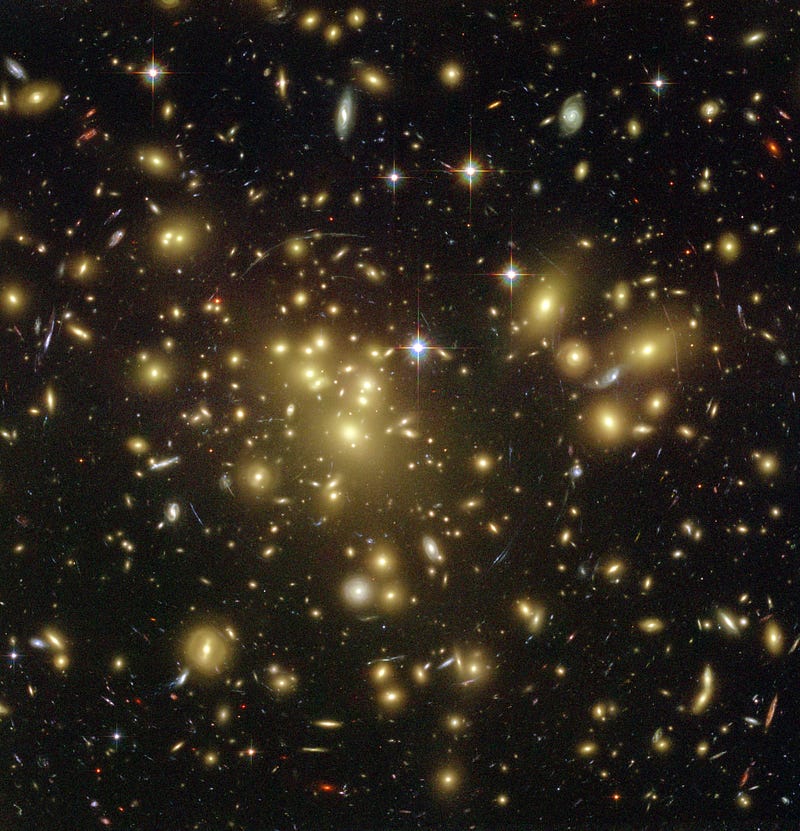
When we add up all the normal matter we know is there in the Universe — things like protons, neutrons, electrons, and photons (radiation) — there’s plenty of it present: some ~10^80 protons and electrons apiece, with slightly fewer neutrons, and about a billion times as many photons on top of it. But there isn’t enough to account for the mass that we see in the Universe at all; we need about five times as much matter in a form that can’t interact electromagnetically the way normal matter does.
That’s what dark matter is. So how would we figure out what its temperature is?
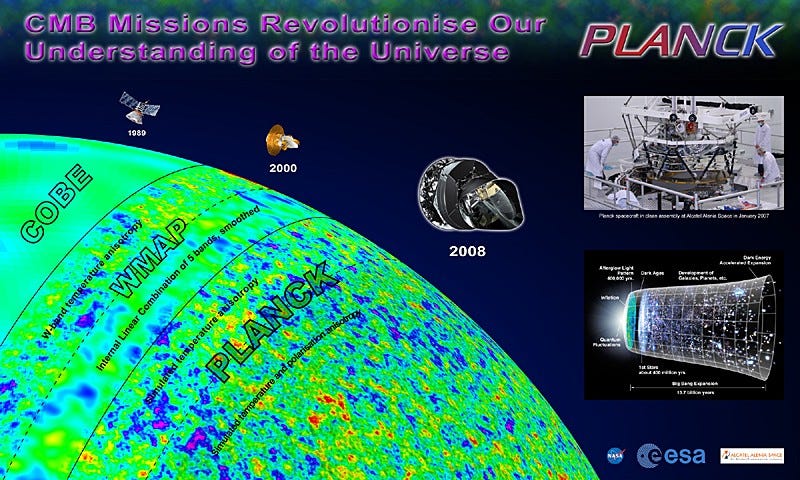
You might think to go back to the earliest stages of the Universe that we can observe: to the cosmic microwave background, or the radiation left over from the aftermath of the Big Bang. That’s not a bad place to start, in fact! When the Universe could first be accurately described by the hot, dense, expanding, cooling, and almost-but-not-quite perfectly uniform conditions we associate with the Big Bang, two competing forces immediately go to work on the largest scales.
On the one hand, all the matter-and-energy in the Universe, roughly evenly distributed, is expanding away from all the other matter-and-energy present. The metric expansion of space works to dilute the energy density of the Universe, and the outward pressure from radiation and other relativistic (close to the speed-of-light) particles works to make it even more uniform, preferentially streaming energy out of the overdense regions.
But on the other hand, gravitation works to preferentially draw more matter into the overdense regions. It’s a runaway process: the more matter you collect in one space, the more strongly it attracts even more matter towards it. So these are the two competing forces at play: the expansion of space and the outward pressure from radiation and fast-moving matter working to slow the growth of imperfections in the Universe, fighting against the attractive force of gravitation on scales small and large.
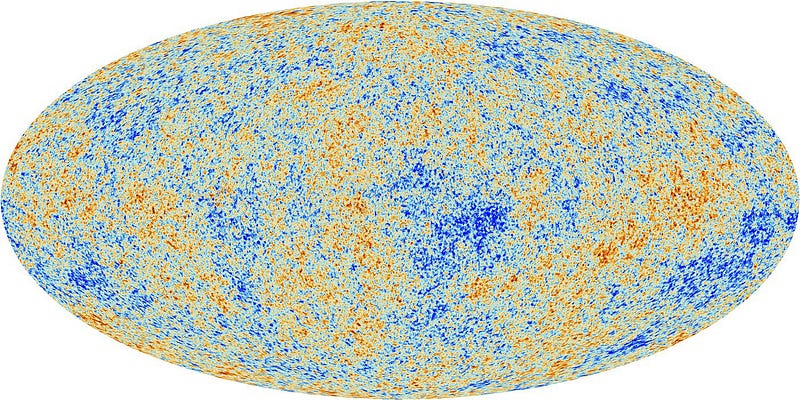
This is the most accurate, comprehensive “baby picture” of the gravitational over-and-underdensities in the Universe: a snapshot from just 380,000 years after the Big Bang. The locations of the greatest overdensities are shown in blue, the underdensities are in red, and yellow represents regions where the density is average. (And where the Universe is very, very boring.)
The way this density map is distributed contains a lot of information, including:
- The sizes of the density fluctuations (how many degrees on the sky they take up),
- The magnitudes of the fluctuations (how many fractions-of-a-degree they are above/below the average), and
- The correlations of the fluctuations (how likely you are to find a hot/cold spot of a certain magnitude near another hot/cold spot of a given magnitude).
When we plot how the density fluctuations are distributed when the Universe was just 380,000 years old as a function of scale/size, this is what we find.
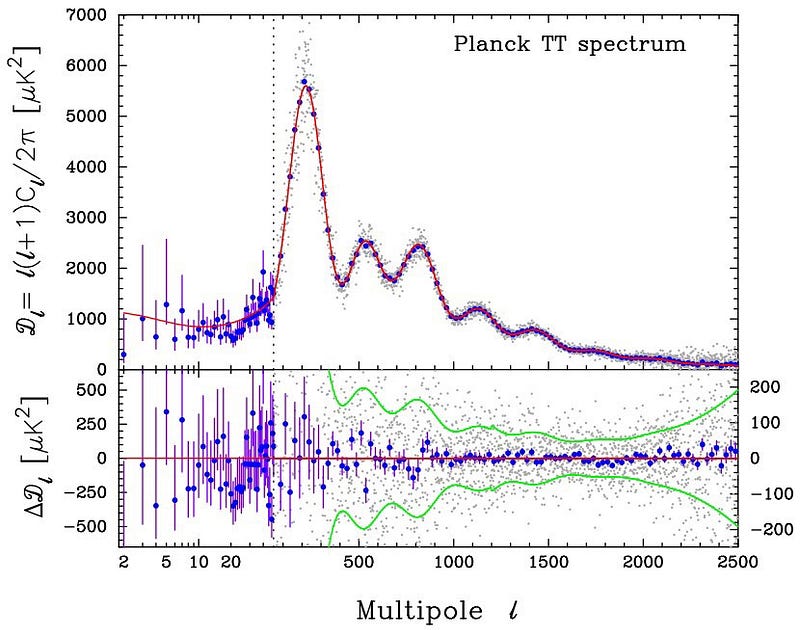
This graph is incredibly useful for telling us things like what the curvature of the Universe is, how much matter-and-radiation are in it, how much of the matter is normal (protons, neutrons, electrons, etc.) versus how much is dark matter, and a number of other things.
But radiation has been too important for too long, and the fluctuations — in terms of absolute magnitude — are still too small for the temperature of dark matter to come into play. So if you want to learn something about the temperature of dark matter, looking at the Cosmic Microwave Background tells you nothing! But all of that begins to change if you’re willing to wait just a little bit longer.
Because now, once the Universe forms neutral atoms, radiation has far less of an effect on how structure grows. Gravitation — particularly in the overdense regions — begins to win. If dark matter was hot, meaning if the particles it was made out of were moving quickly at this time, it would exert an outward pressure and preferentially stream out of the overdense regions, preventing them from growing too quickly. Because the smallest scales are the ones that have the opportunity to gravitationally collapse first (since gravity only travels at the speed of light), a Universe that consisted of hot dark matter would have fewer structures on small scales than a Universe that consisted of colder dark matter.
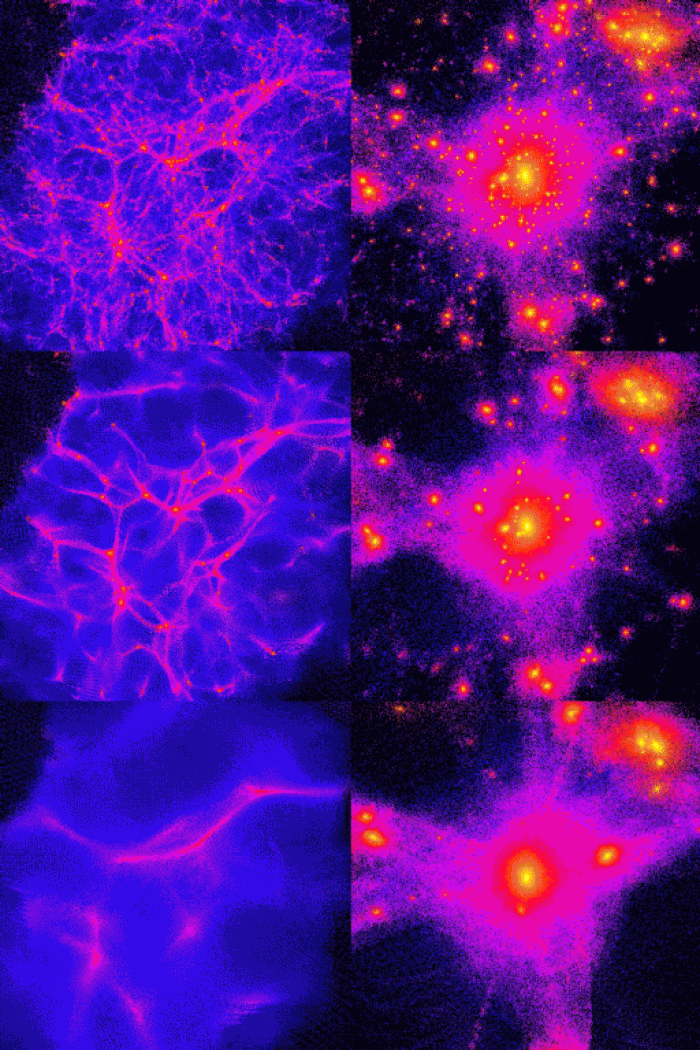
We could simply look at a map of the Universe and eyeball it, but modern cosmology is a much more quantitative science than that! Instead, just like we did for the cosmic microwave background, we can do something very similar:
- measure the magnitude of the over/underdensities of matter in the Universe as a function of scale (by using a tracer, like galaxies),
- measure the likelihood of finding another over/underdensity of a given magnitude nearby, at a certain distance, and
- see how what we observe matches up with theoretical predictions/simulations of a Universe with/without dark matter of a given temperature.
Here’s what theory tells us.
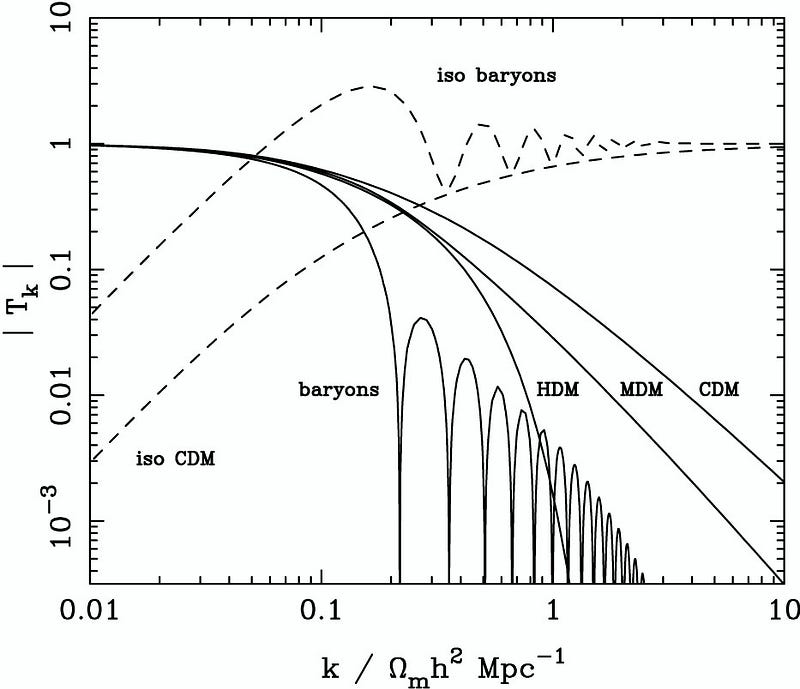
In a Universe with 100% baryons (i.e., with all normal matter and no dark matter), we get these massive asymptotes and wiggles, where the likelihood of a correlation at certain scales drops all the way down to zero.
On the other hand, the dark matter-filled Universes (i.e., with 100% dark matter) are totally smooth and wiggle-free, but either have a cutoff on small scales (for hot dark matter), a quantitative drop in scale (for a mix of hot-and-cold dark matter), or no drop at all (for cold dark matter alone).
It’s 2014, and the best measurement we have of this type of data — known either as the matter power spectrum or the transfer function, depending on how it’s presented — comes from the Sloan Digital Sky Survey.
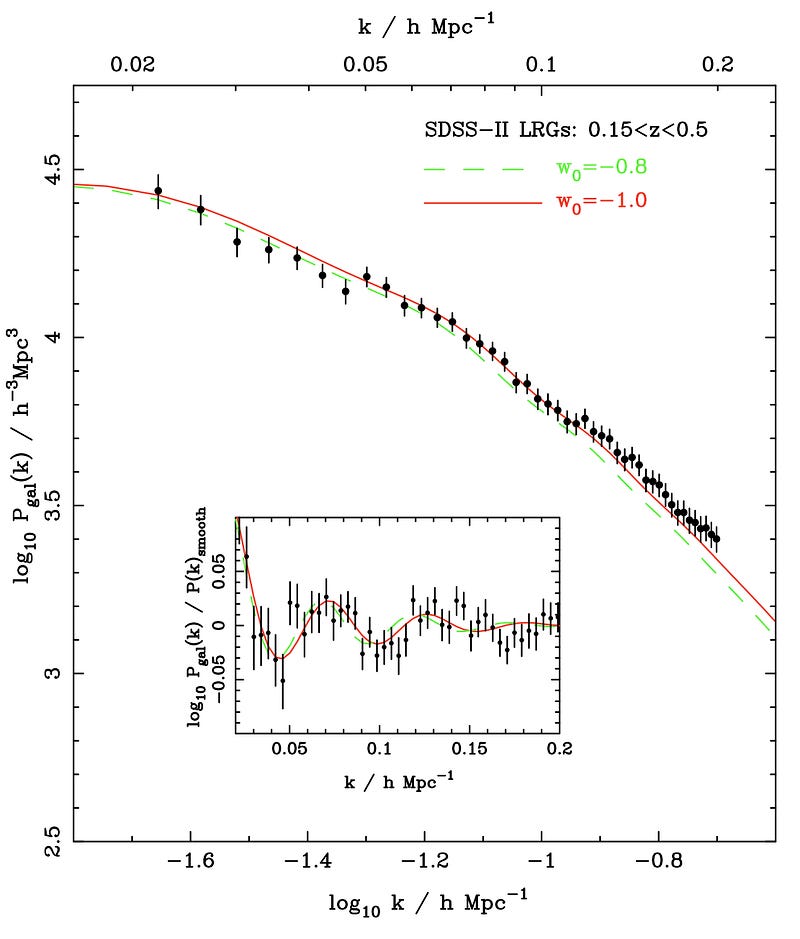
The little wiggles we see tell us that the Universe is — in terms of matter — about 85% dark matter and 15% normal matter, but that there is no cutoff or drop on small scales. In other words, as best as we can tell, well over 95% of the dark matter is cold, or was moving very slowly at all times.
What this means is that if this dark matter was ever in thermal equilibrium, or was ever moving quickly like the other particles shortly after the hot Big Bang, it has to be massive enough so that it slowed down to extremely non-relativistic speeds when the Universe was very young. There’s even one more thing we can look at to measure just how cold this dark matter had to be: the Lyman-alpha forest.
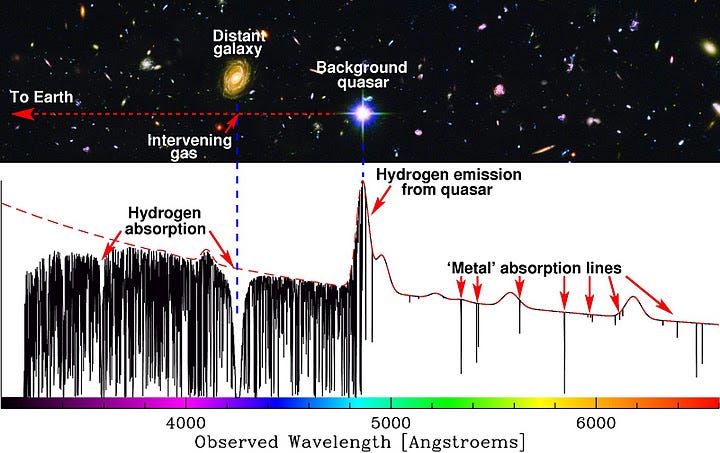
When we look at a very distant emission source — something like a quasar — it emits a wide, broad spectrum of light. But along the way, that light gets absorbed by all the intervening clouds of gas along the way.
How “collapsed” those gas clouds are tell us something about how structure has formed on the smallest scales; if the dark matter were warmer, the depths of those lines would be suppressed by a specific amount, while if the dark matter was colder than a certain amount, those absorption lines would be up to 100% efficient. So what do we see?
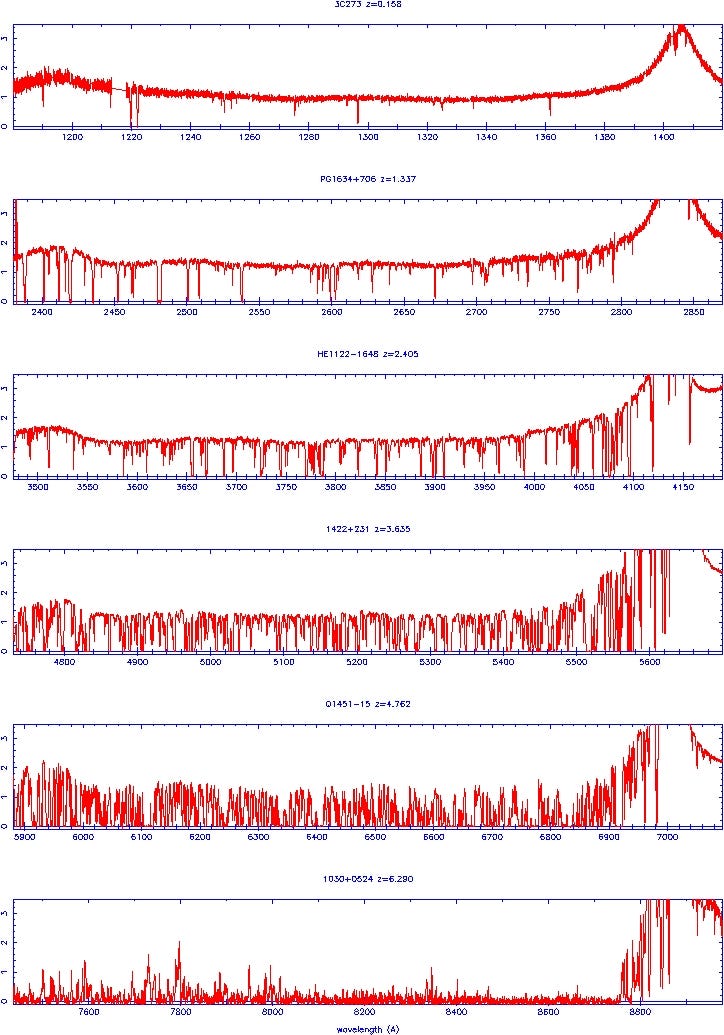
As far back as we can look, these intervening, ultra-distant clouds of hydrogen gas teach us that, if there is dark matter, it must have very little kinetic energy. So this tells us that either the dark matter was born somewhat cold, without very much kinetic energy, or it’s very massive, so that the heat from the early Universe wouldn’t have much of an effect on the speed it was moving millions of years later on.
In other words, as much as we can define a temperature for dark matter, assuming it exists, it’s on the cold side.
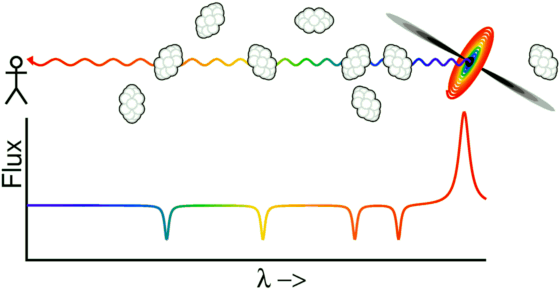
And that’s how we know the temperature of dark matter: from structure formation and from intervening clouds of hydrogen! So I’m sorry to you neutrino fans who were hoping that the lightest, most elusive of all the standard model particles might also be the dark matter; the neutrinos of the standard model would have been hot, and dark matter is not! A little more complicated than dropping food coloring in water, but if you want to come up with an alternative to dark matter, this is a challenge that no alternative has ever risen to.
The search for dark matter — or a viable alternative that addresses these points — continues.
Enjoyed it? Leave your comment at the Starts With A Bang forum on Scienceblogs!