The Most Wanted Particle
What the world’s most powerful collider found, and may yet still find.
“Innovation is taking two things that already exist and putting them together in a new way.” –Tom Freston
In this sense, the Universe is — quite spontaneously — the ultimate innovator. For everything that exists was put together from a hot, dense, chaotic state where only fundamental, individual and massless particles (and antiparticles) once existed in great abundance.
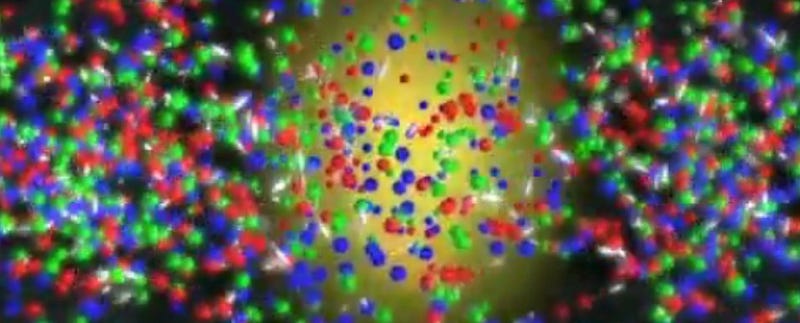
The story of how we went from that state to the one we’re currently in, one where we live in a Universe that:
- is full of matter and not antimatter,
- is littered with stars, galaxies, clusters, and vast cosmic voids,
- contains hundreds of different atomic nuclei that bind together into billions of molecular configurations, and
- gave rise to unimaginable complexity, naturally, including the diversity of life that arose on Earth,
is the most remarkable story that’s ever been told. It’s the story of the Universe itself.
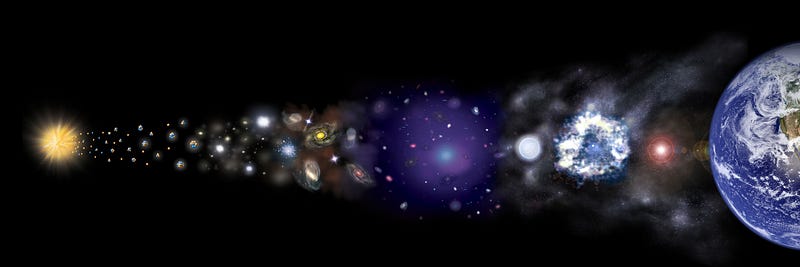
With all of that said, it’s important to recognize that these huge riches the Universe serves us up with all come from just a few simple laws and interactions — the strong, weak, electromagnetic and gravitational forces — and seventeen fundamental particles that come in a few different varieties, if you include their color charge and their antiparticle counterparts.
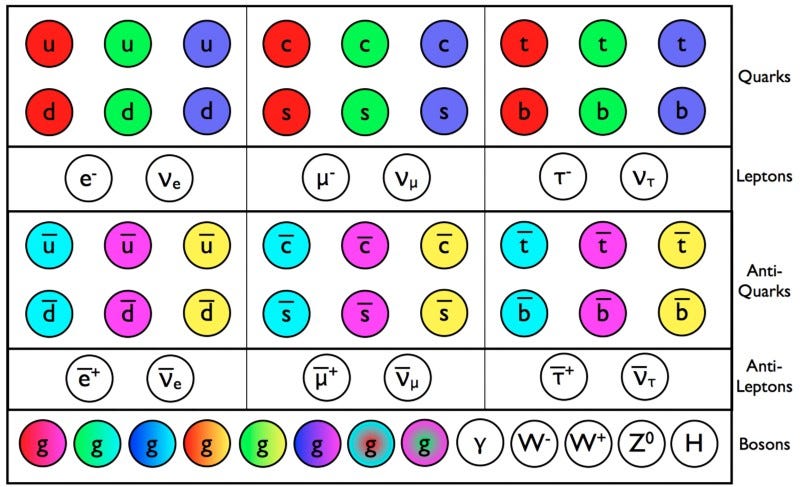
It’s only with the advent of the Large Hadron Collider (LHC) that we’ve found the last and most elusive one: the Higgs boson. It was a tremendous international effort to do so, and the last undiscovered particle in the Standard Model. It was no given that it would exist, either, as it’s the only particle of its type: a fundamental scalar with zero spin. Yet, we know the Standard Model can’t be the whole story of the Universe; there are more unsolved mysteries out there. Hopefully, the restart of the LHC, along with the ensuing higher energies, will help us answer some of them.
So how did we get here, and what are we looking for next? I’m pleased to announce, after the success of our last live-stream from Perimeter Institute, that Starts With A Bang will be hosting and exclusively live-blogging a public talk by Jon Butterworth on “The Most Wanted Particle.”
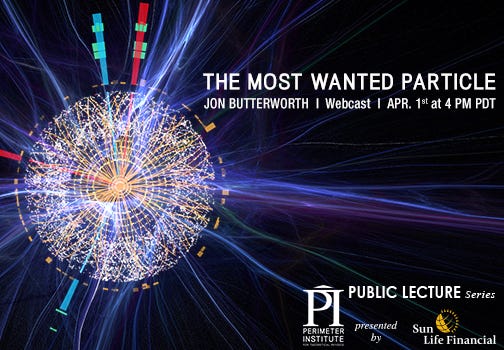
Jon is a fantastic scientist who works on the ATLAS experiment at CERN, a professor at University College London, a passionate science communicator, and should be an informative delight to listen to and watch.
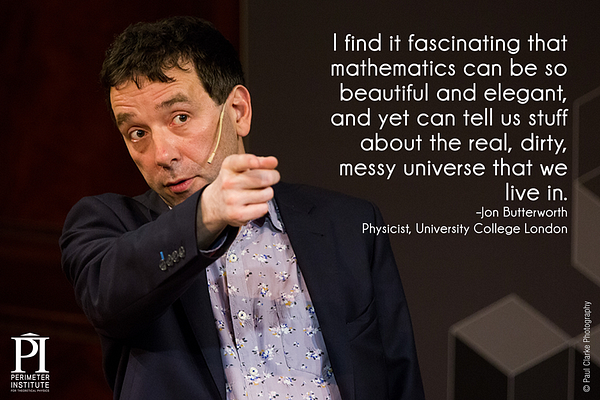
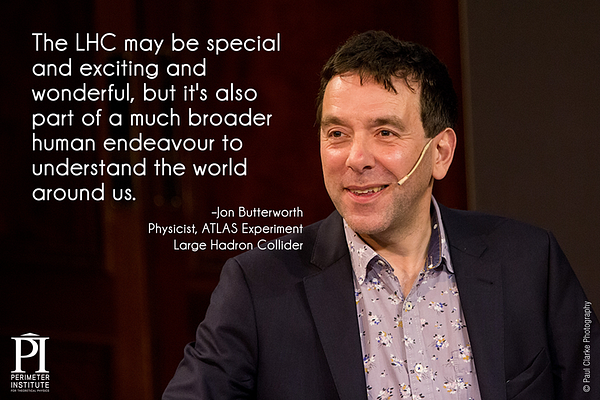
If you want a preview, here’s a trailer for the talk, here’s Jon talking about colliding particles, and here’s Jon talking about the Higgs discovery itself.
So how can you watch the talk and keep up with the live blog simultaneously? Update post-talk: now that the talk is over, just watch is below, and note that the live-blog times correspond to 4:00 PM being the start of the talk!
https://www.youtube.com/embed/zaIa7DWK3o8
Let’s begin the live blog!
Update, 3:45 PM: I hope everyone’s done a good job avoiding the April Fool’s Day shenanigans, the one day I encourage everyone to avoid the entire internet. But welcome to the live-blog of Perimeter Institute’s hosting of Jon Butterworth’s talk on The Most Wanted Particle, which I hope is not only about the Higgs Boson, but what physicists really want the most: the potential discovery a particle that isn’t in our Standard Model!
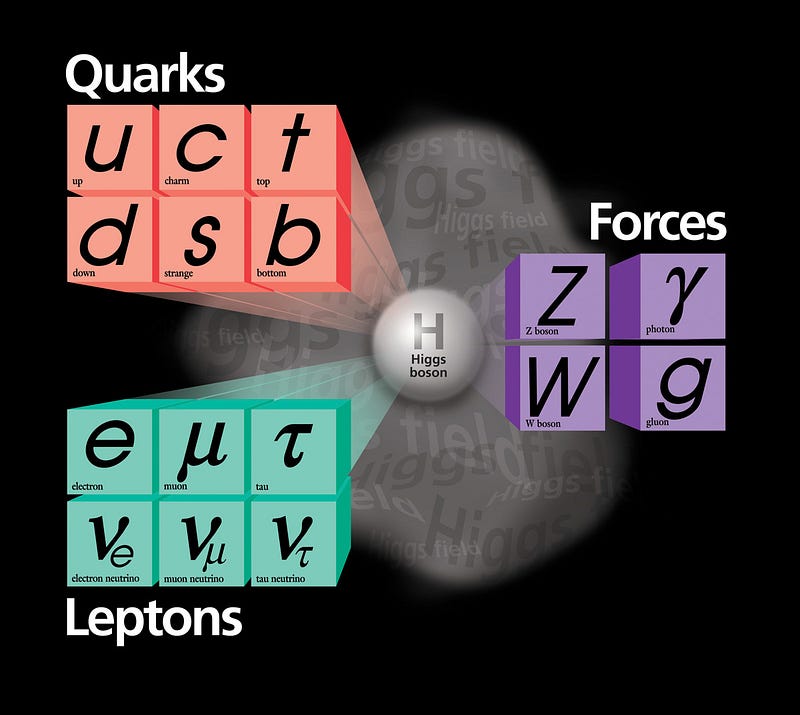
We’ll find out soon!
Update 3:50 PM: Reminiscing about the initial announcement of the discovery of the Higgs boson by both main collaborations (ATLAS and CMS) at the Large Hadron Collider.
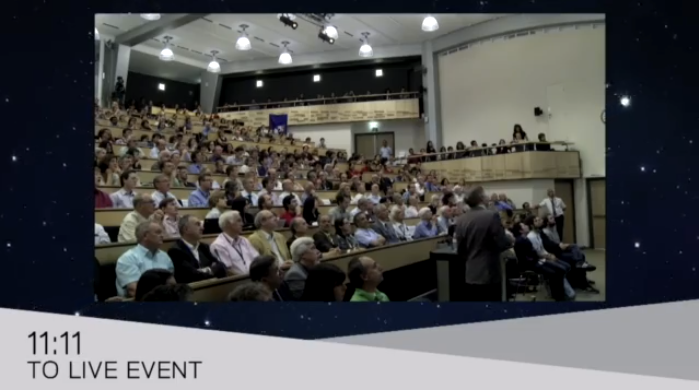
ATLAS went first, announcing a discovery of a new massive, chargeless scalar boson at 126 GeV with 4.9 sigma significance, with CMS going next and announcing the same thing at 125 GeV with 5.0 sigma significance. It was a watershed moment, and the first verified detection of the Higgs boson. Interestingly enough, with the discovery firmly in hand, we can go back to our old data and find that the first Higgs boson produced in a collider was probably created back at Fermilab all the way in 1988! But you need statistics to prove a detection, and it was only in 2012 that we got there.
Update 3:55 PM: Going into the talk, we know there is a new particle at 126 GeV (plus-or-minus 1 GeV or so), but is it really the Higgs? It would have to be spin-0 and have exactly the decays in the right ratios that the standard model predicts. It would have to be the only Higgs, as some variants predict many others. And it can’t be a composite particle.
Do we think all of these things are true? Yes, but we need the LHC and increased data, statistics and more to know for certain. Sometimes, the greatest discoveries come about from unexpected serendipity. Stay tuned.
Update 3:58 PM: Don’t think the Standard Model is definitely all there is, either. There are plenty of things we don’t yet understand, including why neutrinos have mass (and why they have the masses that they do), why there’s no strong-CP violation like there is in the weak sector, why there’s such a large (6 parts in 10^10) matter-antimatter asymmetry in the Universe, and why the masses of all the particles are so much lower than the Planck scale. The Standard Model explains none of these, and — if we’re lucky — the answers to these questions may also turn up, or hints of the answers may turn up, at the LHC in the next couple of years.
Update 3:59 PM: ARE YOU NOT EXCITED YET?!
Update 4:01 PM: It begins!
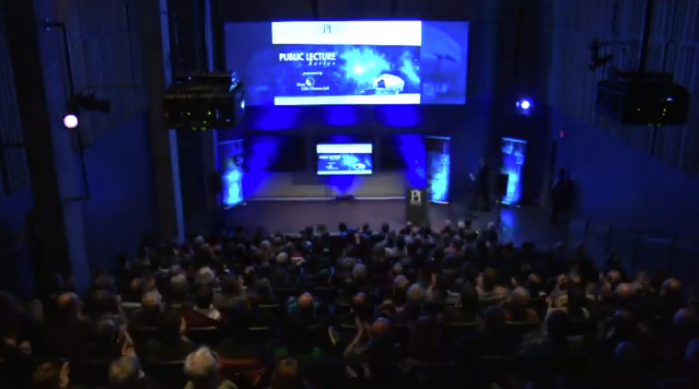
Be active online asking questions and using hashtags; so nice to hear the introduction encouraging that. Even better to hear that they’ve got the audio worked out!
Update 4:03 PM: Jon Butterworth about to begin; was just awarded the Chadwick prize. For those who don’t know, Chadwick discovered the neutron, proving that there were more than just protons and electrons making up atoms and the matter we’re all familiar with. In a real way, it was the first vital piece of experimental evidence that led us away from “atoms” and towards the Standard Model.
Update 4:05 PM: The pictures he shows of the LHC from the air are so different from the picture of the previous record-holder in energy (and my first physics employer in 1997): Fermilab.
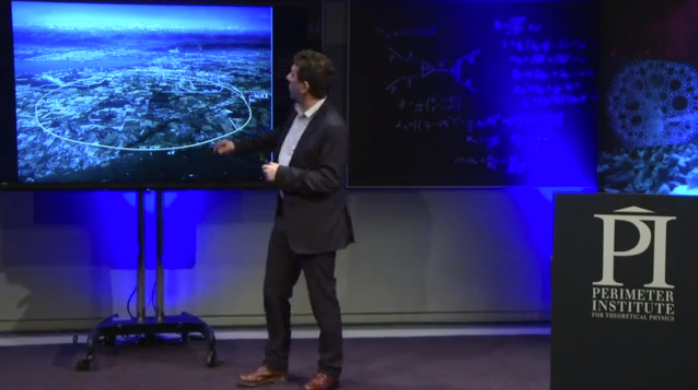
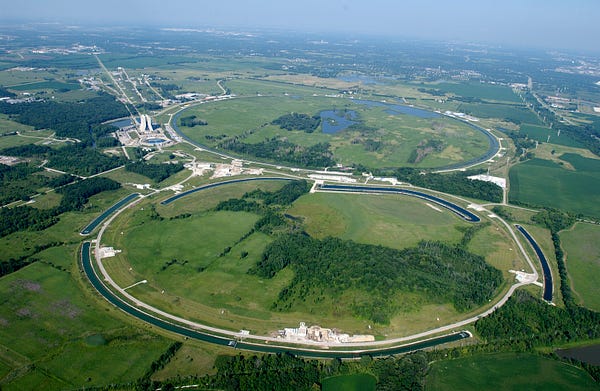
Note that you can’t see where the LHC is from the air; they made the decision to use otherwise unused land to build Fermilab so they could denote the presence above-ground. The LHC is totally underground, so we have to “draw” an imaginary line to visualize where it is.
Update 4:10 PM: Butterworth talks about the limits of how energetic a particle can get, and it’s only determined by two things: the magnetic field you apply and the size of the ring. For those of you wondering why we don’t use electrons instead of protons, which would be individual (clean) particles instead of composite particles (made of quarks and gluons), if you get a particle moving with a large enough energy-to-mass ratio, it starts to spontaneously emit radiation when it’s bent by a magnetic field: synchrotron radiation.
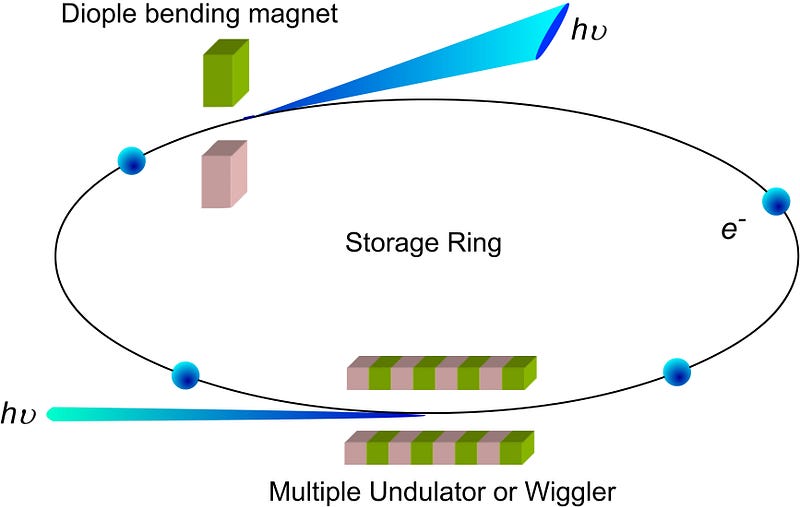
Because protons are 1836 times heavier than electrons, these effects are negligible at the LHC. But with the same size and strength equipment, electrons and positrons would be capped at an energy of about a factor 100 smaller than the LHC will achieve this year.
Update 4:14 PM: Interesting fact: most of the protons going around in this ring miss each other, collisions are relatively rare.
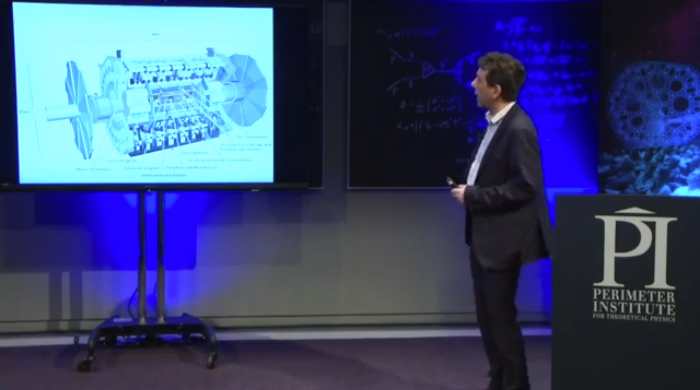
What’s even crazier? The collisions that do occur are so frequent — every 90 nanoseconds — that the speed of light means we physically can’t record all the data! All we can do is reject 99.9% of the data right out as “uninteresting” and trigger recording for the most interesting 0.1%, and even then we can only write down about 0.1% of that data that passes certain tests. So right away, we’re throwing away 999,999 out of every 1,000,000 collisions.
Luckily, we’ve explored “most” of what comes out very well at other, lower energy colliders in the past. It’s only the newest, most energetic stuff that’s going to push the frontiers of physics back.
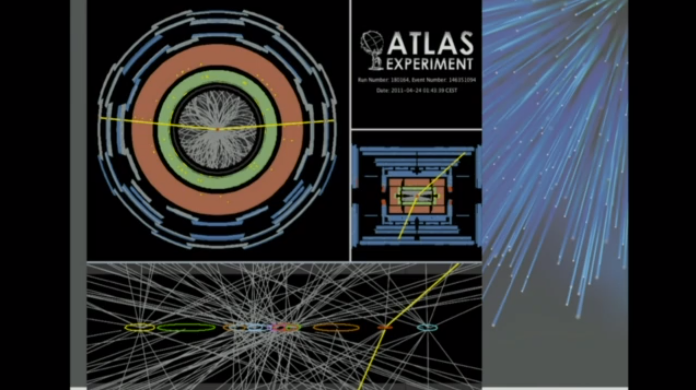
Update 4:18 PM: Why do muons make these long, straight tracks where no other particles do? Three reasons in combination:
- They’re long-lived; of all the unstable particles, neutrons live for 15 minutes, but muons are the second longest lived at about 2.2 microseconds. That’s long when you move close to the speed of light!
- They’re heavy compared to electrons: 206 times heavier. (Same as the number of bones in the adult human body.) So while electrons bend severely in the detector’s magnetic field, muons don’t.
- And finally, its cross-section with matter is small, unlike protons, neutrons, pions and other baryons and mesons.
So that’s why you need these big muon detectors far away from the collision point.
Update 4:25 PM: Simple but profound: why go to high energies with our accelerators?
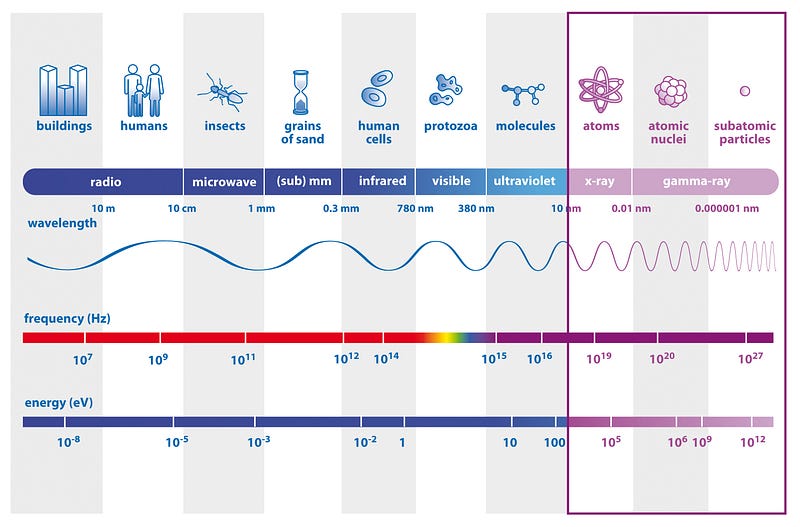
Because it takes shorter and shorter wavelengths to see smaller and smaller things. Just like your eyes are great for seeing facial features but terrible for seeing atoms, low energies are great for probing atomic physics but terrible for probing subatomic particles. To get to the smallest, most fundamental particles, we need to go to higher energies.
Update 4:26 PM: The “Zed” boson. Oh Strong Bad, how I miss your “zee” vs. “zed” jokes.
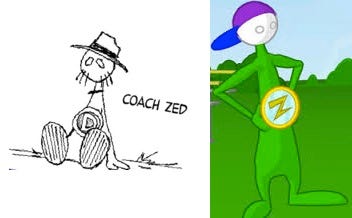
Update 4:33 PM: What is the Higgs field? He finds an interesting analogy from condensed matter physics: imagine an ordered set of magnetic dipoles (North-South poles) on the left, versus an unordered, random one on the right.
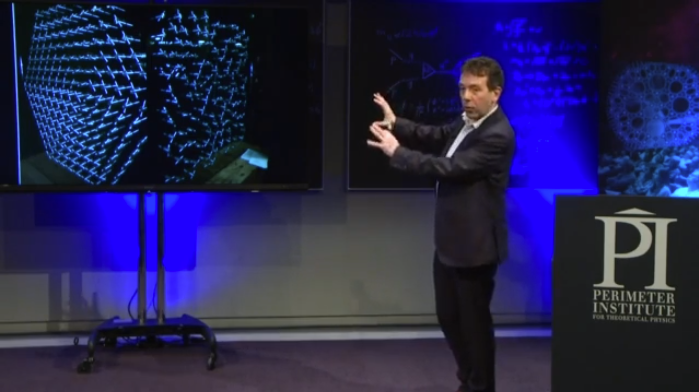
The one on the right is more symmetric, surprisingly: it’s roughly the same from all directions. But there are only specific directions that the one on the left looks the same, and that’s the one that the Higgs field is more like: if you make a “ripple” in one bit of that field, everything else responds to it. Whereas on the right, it would still look just like a random mess.
Update 4:40 PM: Very abstract to bring in Feynman diagrams and quantum field theory here, but he’s trying to explain how you make a Higgs boson in the first place, and the fact that if you smack an electron and a positron together, they can not only interact electromagnetically, but can interact via the weak interaction, and specifically via the Z-boson. (Zee from me, Zed from a Canadian.)
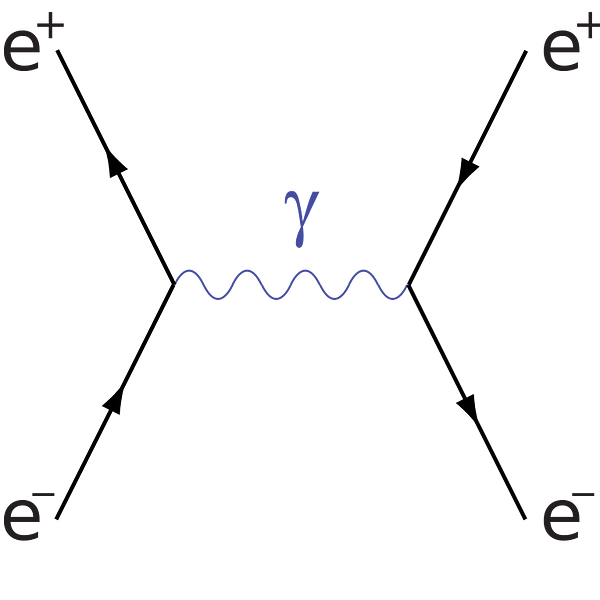
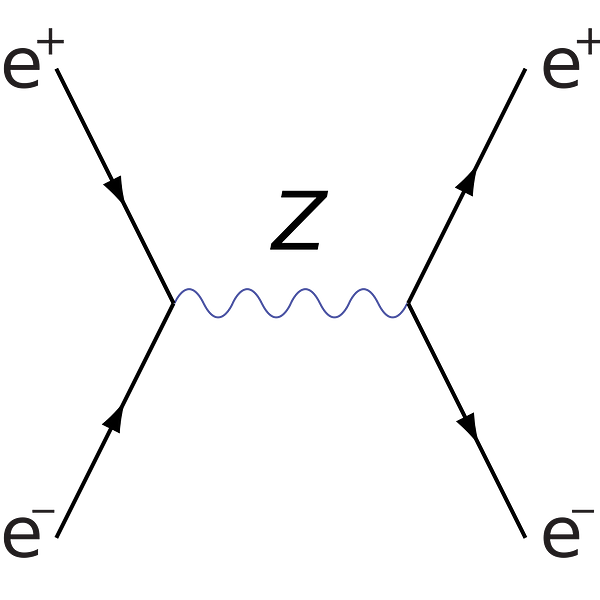
But the Z-boson is massive, while the photon is massless. So what happens? If you collide an electron and a positron at the right energy — at around the mass of the Z-boson — you see the impact of having a massive particle there.
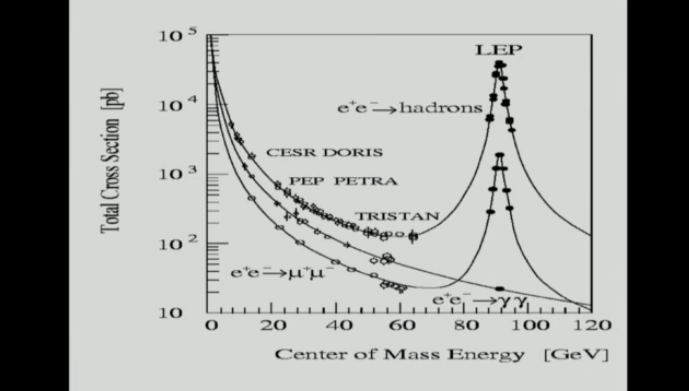
This is the same analogy behind how we try to find the Higgs, and why we’re looking for a “bump” in the different things it can produce.
Update 4:42 PM: So if you get an extra bump in your data at a specific energy, you expect there to be a new particle! It took years to get enough data at the LHC to get this bump.
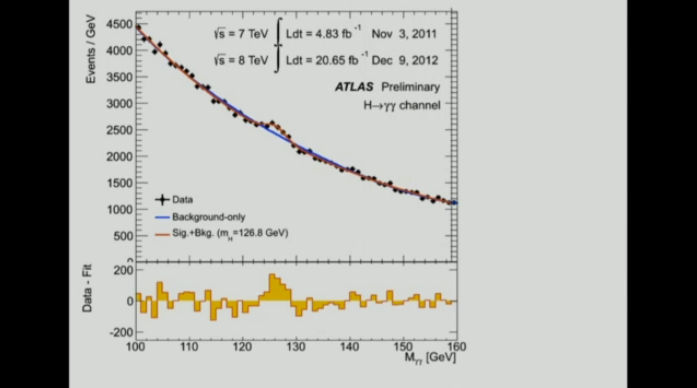
Note all the other minor departures from the “background,” and how much data you need to produce a tiny, tiny “bump” like this.
Update 4:45 PM: Hugely important bit here: Jon Butterworth says the most convincing bit of information was that CMS — the other detector — with completely independent technology and data, found the same signal at the same energy with the same significance. This is how science works: you need independent confirmation to verify that an effect is real, and not an artifact of your experiment. This is why “faster-than-light neutrinos” were never taken seriously, because it could never be confirmed by independent teams, but everyone accepts the existence of this new particle.
Update 4:49 PM: So here’s where I wanted to be: where are we now?! We’ve got all the Standard Model particles, so what’s next? He puts up this nice graphic:
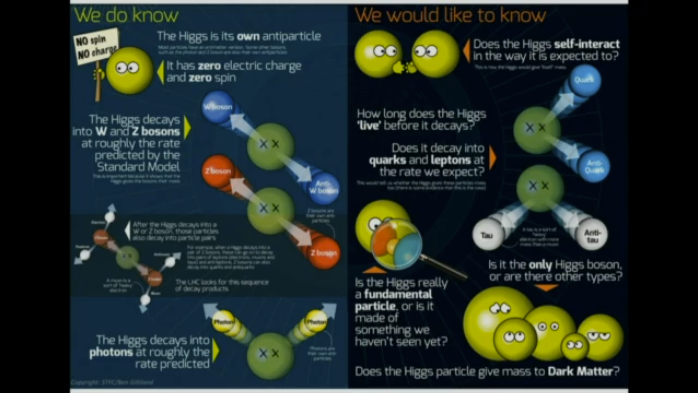
We’re not 100% sure of a whole lot of things:
- the Higgs’ self-interaction,
- the Higgs’ lifetime (it’s very hard to measure lifetimes of 10^-25 s),
- what its decaying branching ratios are (how much it decays into up quarks, downs, electrons, neutrinos, etc.),
- is the Higgs a composite particle (not that we can see, but very hard to probe this; we can only place constraints),
- and are there multiple Higgs particles?
This last one is a prediction of Supersymmetry (SUSY), and if it’s relevant for solving the hierarchy problem (why masses of the Standard Model particles are so much lower than the Planck scale), we should find at least one more at the LHC over the next few years.
Update 4:52 PM: One point he glosses over that’s vital: when the Higgs was first discovered, we hadn’t measured its spin, because we didn’t see certain decays. We saw it decay into two spin=1 particles, but you can have 1+1=2 or 1–1=0, so it could’ve been that this new particle (Higgs boson?) was spin=2 or spin=0. But we’ve subsequently seen it decay into two spin=½ particles, which can mean ½+½=1 or ½–½=0.
Well, if the same thing is decaying into two spin=1 particles and two spin=½ particles, it can only be spin=0 itself, and therefore we know it has the expected properties!
Update 4:55 PM: “Matter-antimatter asymmetry, dark matter, dark energy, unification, hierarchy problem…” these are the unsolved problems he knows need to be solved. Will the LHC provide compelling clues to any of these?
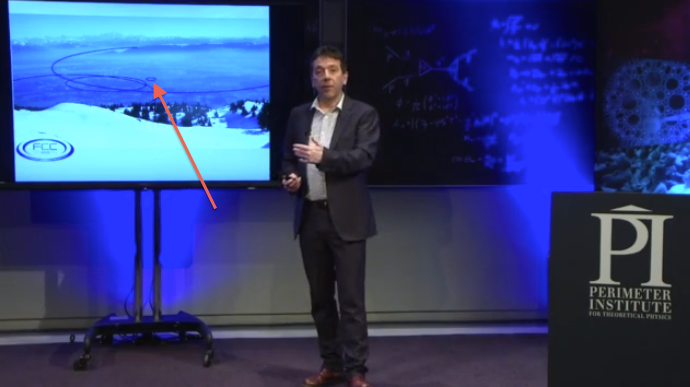
Well, the LHC’s size is represented by the circle shown by the red arrow; other, larger (and hence, more energetic) colliders are proposed. But will they find anything new?
It’s potentially terrifying, but there may be no new particles for many orders of magnitude in energy, and so the Standard Model may be all we find even if we build an accelerator the size of planet Earth!
Update 4:59 PM: We finished on time, and it’s Q&A now. First one: could the LHC produce dark matter? He only talks about the SUSY possibility, which would give you “missing energy”, which is the same thing a neutrino would look like. But if you saw a “bump” in your missing energy spectrum (vs. what you predict for neutrinos only), that would be your evidence.
Update 5:02 PM: What is the origin of electric charge? That’s a good one! He can talk to you about conservation of electric charge, but why is it quantized? Why is it discrete? Why do electrons have charge of “-1” but quarks have fractional charges? And why — under the same rules — are there no magnetic charges? He doesn’t state the “truest” answer we have: we don’t know.
Update 5:03 PM: The evidence for antimatter is overwhelming, in fact, of all the standard model particles that have antiparticles, which is all of the fermions (quarks, charged leptons, neutrinos), we’ve actually directly detected all of the predicted antiparticles.
And that’s it for the talk and the Q&A! Thanks to Jon Butterworth for a great talk; to be fair, he brought us all the way up to the present day limits of our knowledge, I just want there to be more!
Leave your comments at the Starts With A Bang forum on Scienceblogs!