Could “nuclear clocks” drive a technological revolution?
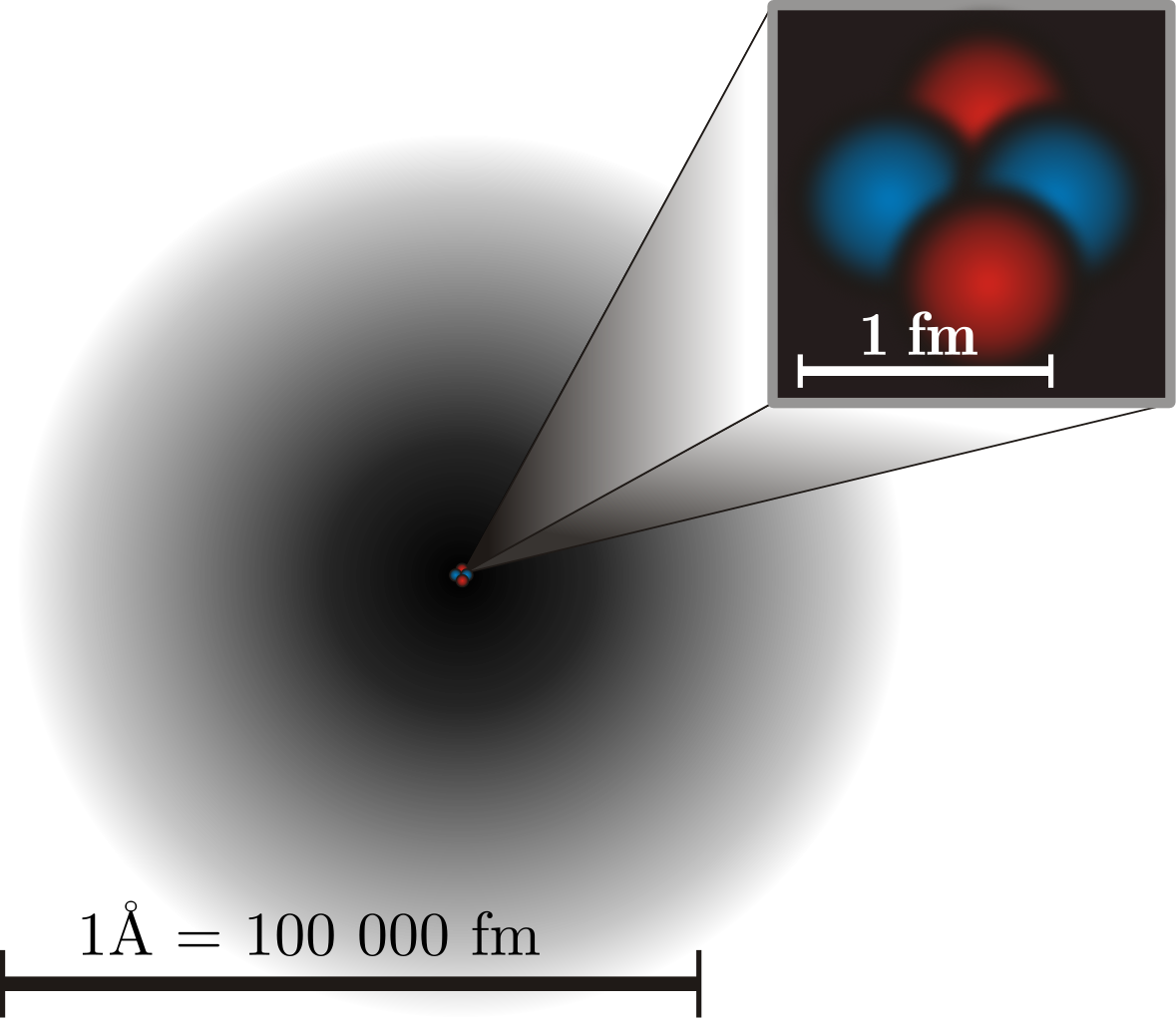
- From the pendulum clock to quartz timepieces to astronomical pulsars to atomic clocks, improvements in timekeeping have brought new science and technology applications along with them.
- Currently, atomic clocks have enabled the most precise timekeeping of all, with accuracies as good as one second every 33 billion years: more than double the present age of the Universe.
- But a new type of clock, based on nuclear rather than atomic transitions, could bring us better time accuracies than ever before, offering 1000% improvements over current technologies. Here’s how, plus what it would all mean.
In all of human history, it’s always been the case that whenever you have a scientific advance, new technological applications and capabilities are certain to follow. Even in a field as mundane as timekeeping, ancient advances from sundials to pendulum clocks to quartz clocks enabled technologies like remote synchronization and reliable navigation across the globe, day or night.
In the mid-20th century, the greatest natural clocks — millisecond pulsars — were discovered, while atomic clocks were developed right around the same time. Instead of keeping time to a second’s accuracy over days, weeks, or even months, we improved to a single second’s accuracy over thousands, millions, and now even billions of years. At last count, optical lattice atomic clocks can keep time to better precision than 1 part in 1017.
Today, atomic clocks play an essential role in telecommunications, financial transactions, computers, GPS satellite navigation technologies as well as a variety of scientific applications. We can synchronize clocks around the globe with ~nanosecond precisions. But still, there are limits to what we can do, and those are set by the physical limits of atoms. Yet there’s a tremendous hope for surpassing all current limits by more than an order of magnitude: nuclear clocks. Here’s the science of how it all works.
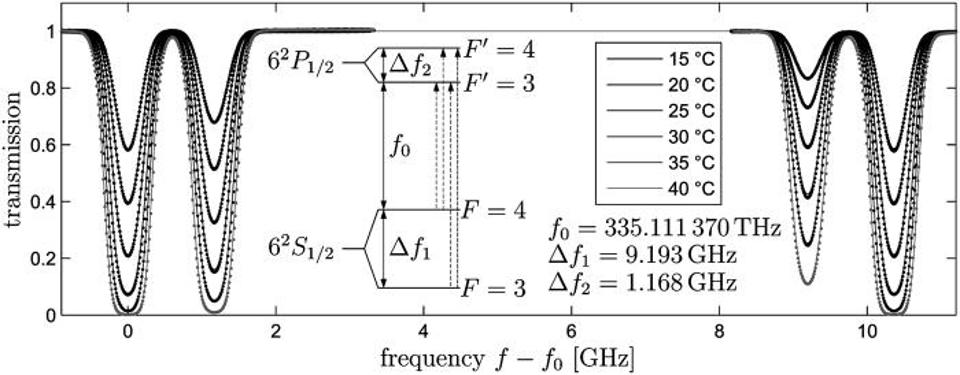
One of the biggest revelations of the 20th century was the notion of relative time. Originally — and for centuries — time was conceived of as an absolute quantity, ticking away at the same rate at all locations everywhere in the Universe. According to the idea of absolute time, no matter where you are or how you’re moving, in every “one second” that elapses for you, that same one second also elapses for everyone else, under all physical conditions.
Only, if the speed of light is a constant for everyone in the Universe, this is an impossibility.
The rate at which time passes, instead, is related to the rate at which one observer, relative to another, is in motion through space. This includes both the simple motion of special relativity, related to your relative velocities, as well as the more complicated motion of general relativity, related to the relative curvature of space (as well as how it changes) between two observers. There’s no such thing as absolute time; the only way to define “time” that makes any physical sense is to pick one frame of reference that all observers agree upon, and then to calibrate your clocks relative to that one particular frame of reference.
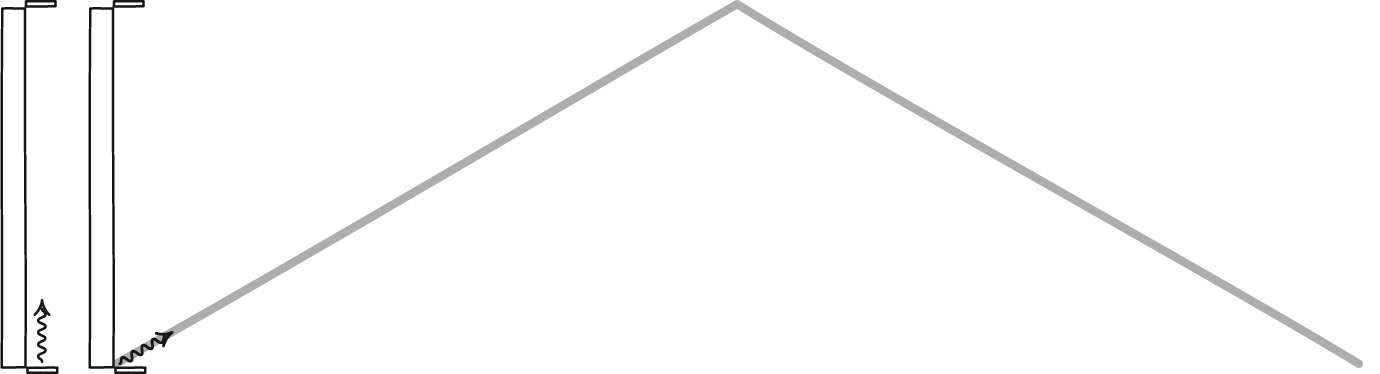
Thankfully, humanity has precisely that: a frame of reference that all humans can agree upon is the correct one to synchronize around: the surface of the Earth itself. Yes, the rate at which time passes will depend on the cumulative motion of the Earth around the Sun, your distance from the center of the Earth, the local amount of gravity beneath your feet, and just how quickly the Earth is rotating beneath your feet.
Still, these are surmountable problems; with enough data points, we can quantify the effects of each and every one of these phenomena. Once we’ve done precisely that, we can “keep time” equally well, everywhere on Earth and beyond it, to the limits of the precision of our timekeeping apparatuses.
However well we can keep time, then, determines what our technological capabilities will be. There are many tasks that require an incredibly accurate knowledge of time, synchronized across many different locations around the globe, and a few even beyond our terrestrial world. Any question you have about “which event occurred first” or “how precisely can we measure the exact moment at which this event occurred” can only be answered as well as you’re capable of measuring time according to the standard-of-rest (or reference frame) that we’ve selected.
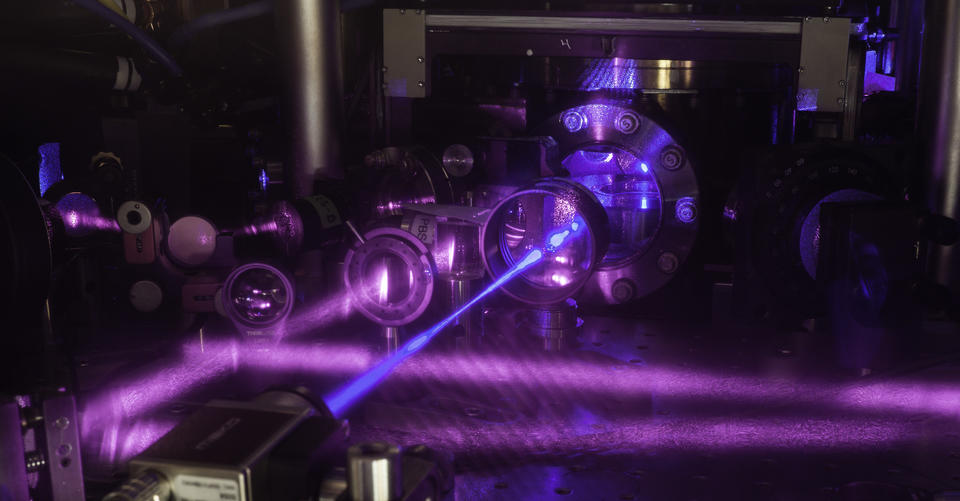
At present, the atomic clock is the best technology we have for measuring time to the greatest precision of all. The way that atomic clocks work is, in principle, very simple.
- You have an atom, or more accurately, a set (or lattice) of identical atoms, that have certain intrinsic properties.
- One of those properties is a series of energy levels, where electrons in a lower energy level can only absorb photons (the quantum counterpart of light waves) of a specific set of wavelengths, and those excited electrons can only de-excite by emitting photons of that same set of specific wavelengths.
- Since atoms are made out of a charged nucleus and a set of oppositely-charged electrons, all of which have an intrinsic angular momentum (spin) and where the electrons are in orbit around the nucleus, the atoms have their own internal electric and magnetic fields.
- These fields then affect those energy levels, causing them to “split” into fine and hyperfine structure.
- These split energy levels have only tiny differences in energy between them, and so these transitions are smaller, more sensitive, and lead to more precisely-measurable timescales.
- And by continuously “pumping” these atoms with a laser, which keeps them in an excited state, we can cause them to emit light of a specific, well-known frequency in a pretty much continuous fashion.
Since we can simply count the number of wavelengths of light that pass by, and the speed of light is constant for everyone, we wind up with a method for calculating “time” that can be made consistent, through a synchronization process, for every observer who possesses such a clock.
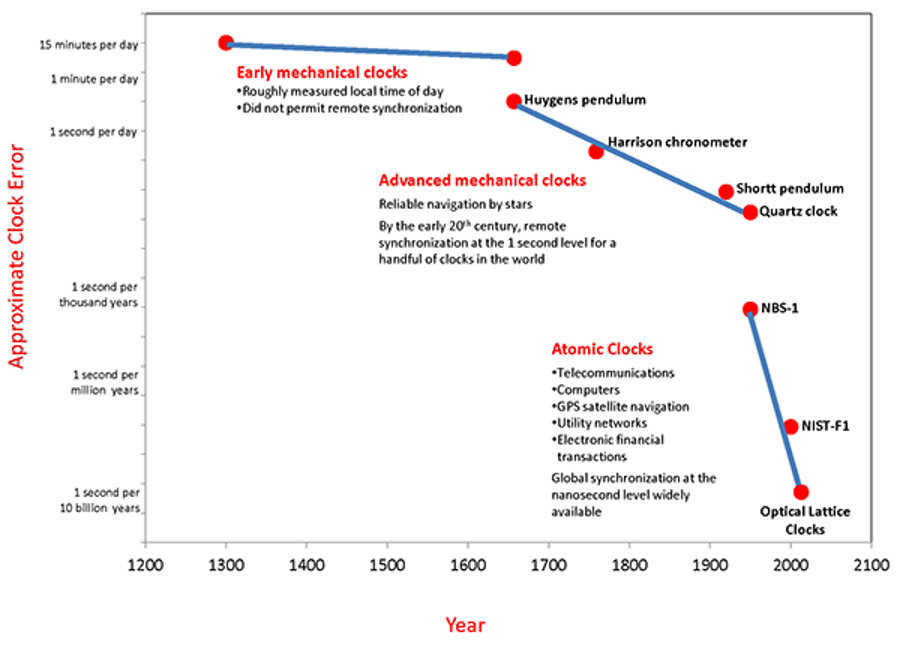
Scientifically, we leverage atomic clocks in a number of ways. There are multiple atomic clocks on board each GPS satellite, and every point on Earth is in constant contact with no fewer than four such GPS satellites at every moment in time. This allows us to “fix” each observer’s location — three spatial coordinates and one time coordinate — to the precision of the atomic clocks on board the satellites.
In the realms of Earth science and climate science, the ability to determine what the influence of gravity is at every point on Earth’s surface — geodesy — helps with everything from understanding Earth’s water cycle to monitoring climate change to monitoring coastal changes and much more.
And, perhaps most fascinatingly, atomic clocks have been hooked up to an array of different long-wavelength observatories across the globe. When the connected observatories make observations of the same object, they can use those atomic clocks to effectively link — through the process of very-long-baseline interferometry — those various observatories together into an array. With an effective resolution given by the distance between the telescopes, this has been leveraged in just the past few years to yield our very first images of a black hole’s event horizon.
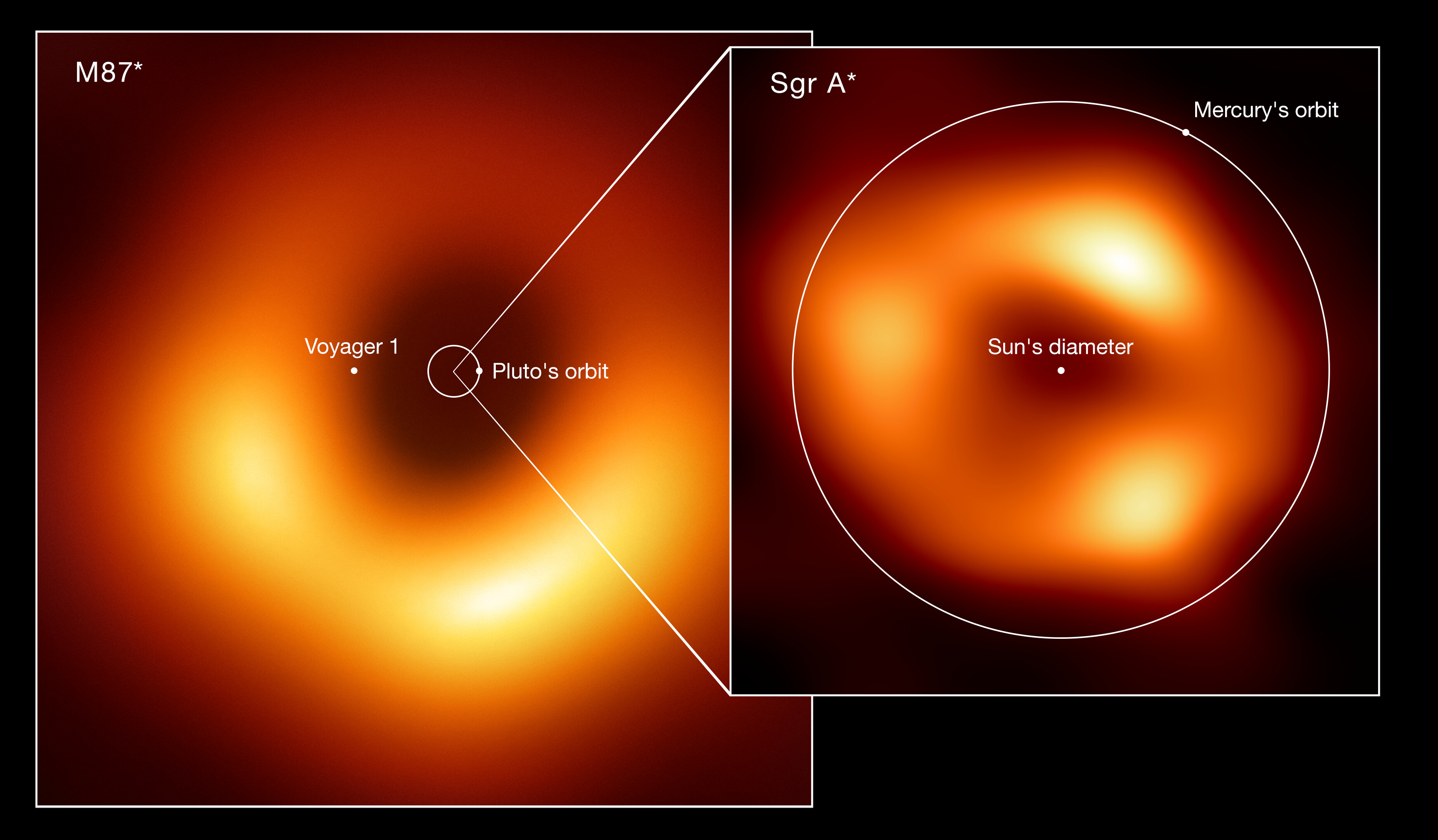
Beyond the fundamental scientific applications of atomic clocks, there are technological ones as well.
- Our ability to measure time precisely determines the precision at which telecommunications between disparate locations can take place.
- It helps synchronize computers around the world at ~nanosecond precision. It helps networks monitor utility usage in real-time across the globe.
- It helps determine the order in which financial transactions take/took place, an essential tool in the global economy.
- It enables GPS users to determine their position on Earth to extreme precision; a timekeeping device that was even a factor of ~10 less precise would result in positions that were ~10 times more uncertain.
- And if you have a device in space, atomic clocks can enable autonomous navigation, rather than having to rely on communications back to a singular reference frame, such as the one we have back here on Earth.
Today, modern atomic clocks can take a variety of approaches, with the optical lattice approach producing the highest degrees of precision. These optical clocks are based on ytterbium or strontium atoms, whereas the older (microwave) standard of cesium atoms, by which the second is still defined, is fundamentally less precise.
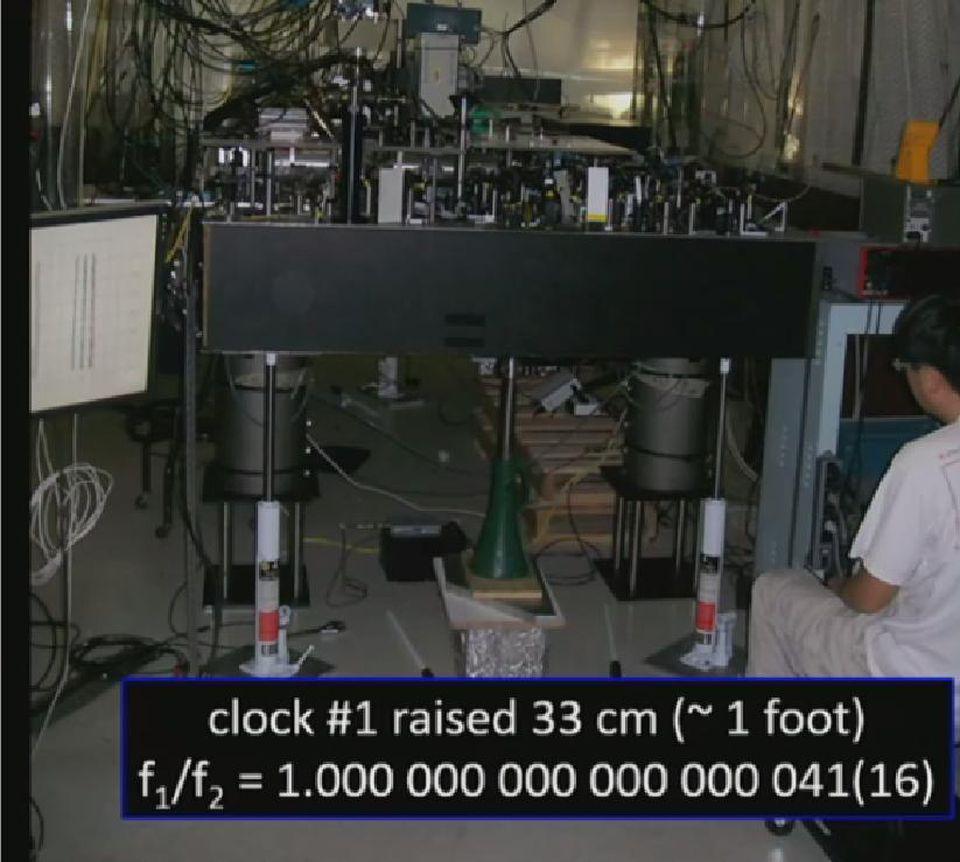
But atomic clocks, even when placed into a lattice and operated at optical frequencies, are still fundamentally limited. Because they rely on electron transitions in an atom’s shell, the benefit of an atomic clock is that the energy of its operation is independent of both space and time; regardless of how fast you’re moving or what type of gravitational field you’re in, atoms exhibit the same quantum mechanical properties. When you excite this atomic transition with (monochromatic) laser light continuously with a laser, you can simply measure how many oscillations of the light have occurred to determine the passage of time.
But atoms are rather large entities; they take up about an ångström, or one ten-billionth of a meter, in diameter. While the internal electric and magnetic fields of the atom determine the fine and hyperfine structure of that atom, there are also electric and magnetic fields present externally, and they perturb these energy levels further. These external fields are the limiting factor for achieving better accuracies with modern atomic clocks, as there is no way to effectively shield the individual atoms from these unavoidable effects.

That’s where, at last, the idea of nuclear clocks comes in. Instead of using the transitions of an electron that take up the space of an entire atom, a nuclear clock relies on a transition inside the atomic nucleus itself to keep time. Just as with an atomic clock, you would “drive” the nuclear transition with a laser of a specific frequency, and then that transition would occur over and over again to lead to the continuous emission of light. Direct laser excitation is a requirement.
A slight difference would be that in order to excite a single atom, you’d have to trap an ionized atomic nucleus; this can be accomplished through a device known as a Paul trap, named after Wolfgang Paul, a physicists completely unrelated to Wolfgang Pauli but remarkable in his own right. You can make a single-ion nuclear clock or, by trapping a chain of multiple ions, a multiple-ion nuclear clock. Of course, atomic nuclei can also be embedded into a crystal lattice environment, similar to how atomic clocks can be. Because the nuclei are so small compared to the atom, and because there are so many of them, these solid-state nuclear clocks offer the potential to compete with the trap-based nuclear clocks at the forefront of this developing technology.
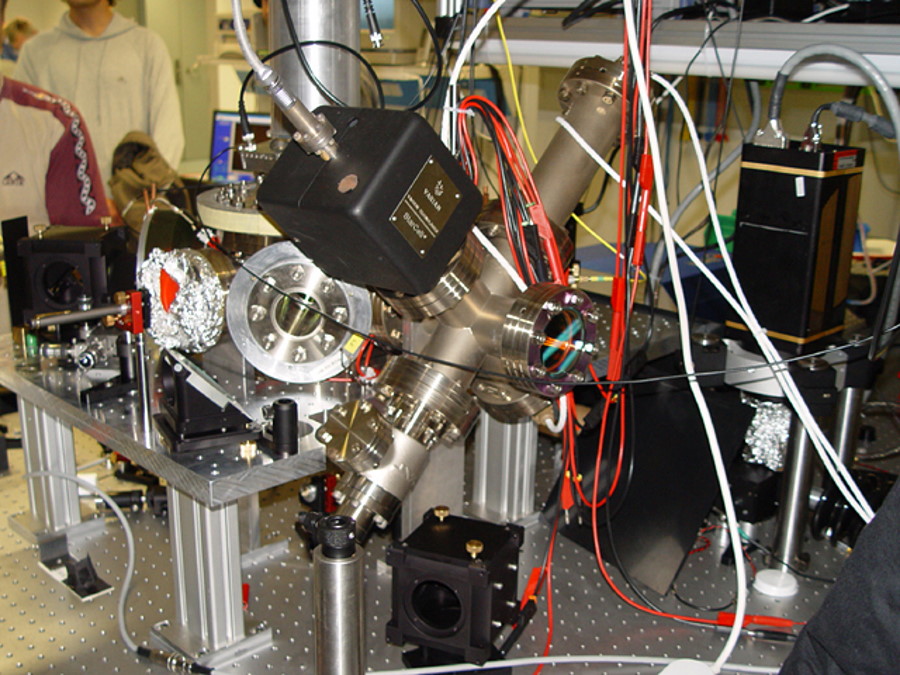
However, unlike an atomic clock, the effect of external electric and magnetic fields is much smaller. In fact, owing to the much smaller size of a nucleus (by about a factor of 100,000), nuclear clocks could lead to much more stringent precisions than modern atomic clocks. Currently, the best atomic clocks are accurate to about 1 second in every 30 billion years, but nuclear clocks could up that to nearly 1 second in every one trillion years, which would be a remarkable improvement.
- Financial transactions could occur with ~picosecond accuracy.
- Global positioning could be achieved with ~millimeter precision.
- The gravitational changes in Earth could lead to us monitoring water-table changes by sub-centimeter levels.
- And radio astronomy, which is used today to image black holes with a telescope the effective diameter of Earth, could be extended into the space around Earth, leading to resolutions that are tens or even hundreds of times greater than at present.
The major difficulty here is that there’s only one known atomic nucleus suitable for a nuclear atomic clock: the excited state of thorium-229. While atomic transitions usually occur on the order of ~1 electron-volt, nuclear transitions are much greater in energy, of thousands or even millions of electron-volts. Thorium-229 is one of only two nuclei known with a transition below 100 electron-volts of energy, and is in fact the lowest: at about 8 electron-Volts. This is a brand new field, and while nuclear clocks do not yet exist, this is the most promising avenue for superseding atomic clocks.
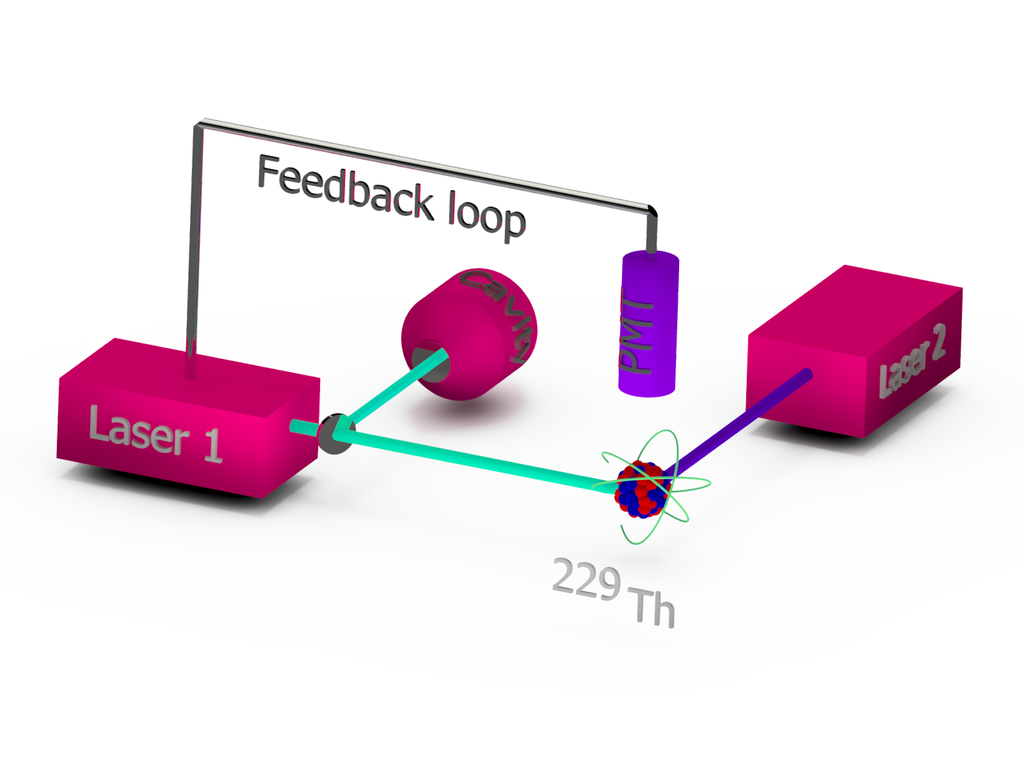
Since the idea of a nuclear optical clock based on thorium-229 was first proposed in 2003, many steps toward its realization have been achieved. Precision gamma-ray spectroscopy experiments have determined the isomeric energy of the excited state with an uncertainty of just 2%. Triply-ionized thorium-229 was successfully trapped into a Paul trap and laser cooled. The thorium-229 isomer was both created and then observed to spontaneously decay back to its non-excited state. And the hyperfine-structure shift, induced by the isomer, was detected for the first time just four years ago.
The stakes are a lot higher, in some ways, than just measuring time to ever-greater precision. If there are rare forms of dark matter or if any of the fundamental constants vary with time, improvements in timekeeping could reveal them. After all, the timescale for light to cross an atomic nucleus is a factor of 100,000 shorter than the time to cross an atom, and so if there are any physical effects that preferentially affect these longer timescales as compared to shorter ones, the difference between atomic and nuclear clocks could reveal them. Although the technology is still being developed, it could be just a few years or decades from now where atomic clocks — like quartz watches and pendulum clocks before them — become more of a steampunk-style novelty than the cutting edge technology they are today.