New USPS Stamp Celebrates Physicist Chien-Shiung Wu, The ‘First Lady’ Of Physics
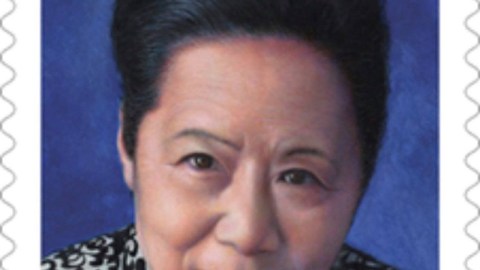
Of all the injustices in Nobel Prize history, her 1957 Nobel snub is the most egregious.
One of the biggest scientific revolutions of the 20th century was the discovery of quantum physics. At the smallest scales, nature didn’t behave like the classical laws of gravity and electromagnetism predicted, but rather started to display bizarre phenomena that clearly obeyed a new set of rules. As we dove deeper into the structure of matter, we discovered the atomic nucleus, made up of protons and neutrons, and a whole plethora of other particles — known today as the baryons and mesons — that are made of the same types of subatomic particles that make up protons and neutrons: quarks and gluons.
But it wasn’t just the structure of matter and the rules that are different between the quantum and classical worlds, but also the nature of symmetries. Classically, we see that matter and light obey the same laws of physics whether you flip directions the same way a mirror does, whether you replace particles with antiparticles (and vice versa), or whether you run the clock forwards or backwards. But in the quantum world, under the right conditions, these can all be violated. On February 11, 2021, the USPS honors the first physicist to experimentally demonstrate that one of these symmetries is violated: Chien-Shiung Wu. Quite arguably, she’s the most deserving physicist never to win a Nobel Prize. Here’s the scientific story of why what she did mattered so much.
In our classical world — the macroscopic, everyday world that describes our common experiences — the laws that govern nature don’t appear to care about a large variety of properties. The laws of physics here are the same as the laws of physics anywhere else; that means they’re invariant (they don’t change) under spatial translations. The laws of physics are also the same now as they are at any other time; that means they’re time-translation invariant. They’re also invariant under boosts, which means you can move at any speed you like and the laws are the same, which is a key component of relativity.
But the three symmetries we talked about earlier all have names, and they’re all (today) known to be violated by one and only one force of nature, the weak force. In particular, these symmetries are:
- Parity (P) symmetry: where you reflect your system in a mirror, and see if it obeys the same rules.
- Charge conjugation © symmetry: where you replace every particle with its antiparticle counterpart, and every antiparticle with its particle counterpart.
- Time reversal (T) symmetry: where instead of running the clock forwards, you run it backwards, checking if the rules are the same.
In the picture above, you can’t tell whether the ball is moving to the right and losing energy with every bounce, or moving to the left and getting “kicked” to higher energies with every bounce. The laws are the same forwards and backwards.
But just as some of the 26 capital letters in the English alphabet obey parity symmetry and others don’t, it’s possible for some of the laws of physics to not obey this symmetry as well. However, beginning in the 1920s, parity did appear to be conserved in every physics experiment ever performed. When any object falls in a gravitational field, either terrestrially or celestially, parity is conserved. When an electron absorbs or emits a photon, parity is conserved. And when any particles collide, scatter off each other, combine, or explode, parity is still conserved.
However, every once in a while, an unstable particle will undergo a radioactive decay. This isn’t a gravitational or an electromagnetic interaction, but a wholly new type of force on display: the nuclear forces. It turns out there are two types of nuclear force:
- the strong nuclear force, which holds protons, neutrons, and all quark-containing particles together,
- and the weak nuclear force, which allows one type of quark to transmute into a different type of quark, sometimes involving leptons/antileptons or additional quarks/antiquarks as well.
Understanding which forces are at play in which interactions teaches us what we should expect to occur.
There are three fundamental classes of radioactive decay (technically there are more, but this is good enough for our purposes), and they rely on the interplay of different forces to drive these decays.
- Alpha decay: this is the most common type of radioactive decay, and occurs when a heavy, unstable nucleus spits out an alpha particle, which is actually a helium-4 nucleus, made of two protons and two neutrons. This decay occurs as a combination of the strong nuclear force (which causes protons and neutrons to attract at very short distances) and the electromagnetic force (where like charges repel), where the products are more energetically stable than the initial nucleus.
- Beta decay: the second-most common type of radioactive decay, this typically occurs when a down quark — the second-lightest quark in the Standard Model — decays into an up quark, producing an electron and an anti-electron neutrino in the process. This is a decay that operates purely through the weak interaction, and before quarks were discovered, was understood to be a neutron transmuting into a proton, releasing an electron and “missing energy” until the (anti-)neutrino was discovered in 1956.
- Gamma decay: this is a purely electromagnetic decay, and occurs when a heavy, unstable nucleus rearranges the particles inside, emitting a high-energy photon and de-exciting the nucleus to a lower-energy state.
Alpha and gamma decays always conserve parity, but beta decays do not.
This was Chien-Shiung Wu’s area of specialty: the study of beta decay in nuclear physics. Originally planning to emigrate from China to the United States to study at the University of Michigan (where she had been accepted), Wu visited UC Berkeley in San Francisco, where her ship arrived in 1936. After touring the radiation laboratory there — and after hearing a story about women being unable to use the front entrance at the University of Michigan — Wu chose to study at Berkeley instead. Working with Ernest Lawrence and Emilio Segrè, she graduated in 1940, working on various aspects of nuclear physics and what we would today call particle physics.
Frustrated with her inability to find a faculty position at a University, she remained at Berkeley as a post-doctoral fellow for a couple of years, finally landing a faculty job at women’s-only Smith College. Again frustrated, as she had no opportunities for research, she joined the Manhattan Project at Columbia University in 1944. Although her task was to develop instruments for detecting radiation, she was contacted about an unexpected and repeated nuclear reactor shutdown. Wu’s research under Segrè, involving radioactive properties of xenon-135 — which undergoes beta decay — was the key to understanding why the reactor was shutting down: the isotope, produced by nuclear fission, was an excellent absorber of neutrons.
The still-unpublished draft of Wu’s paper unlocked the solution to the problem, and helped land Wu a permanent position as a research professor at Columbia after the end of World War II.
In the 1950s, two theoretical physicists — Tsung-Dao Lee, a friend of Wu’s, and Chen Ning Yang — were puzzling over two different particles that seemed to be identical in every way but one: the Theta (Θ) and Tau (τ) particles. They had the same mass, the same charge, the same spin, and the same lifetime. They had a property that we called “strangeness” back then; today we understand that it means each of these particles contained a strange quark. But the one difference was significant:
- the Θ particle always decayed into two pions, a positive and a neutral one,
- while the τ particle always decayed into three pions, two positive and one negative one.
This brought up a big question: were they the same particle or not?
The problem is that parity is a multiplicative quantum number, and the parity of a pion is -1. If you decay into two pions, your parity has to be +1, because (-1)² equals +1. But if you decay into three pions, your parity has to be -1, because (-1)³ equals -1. It led Lee and Yang to put forth the idea that maybe, for the weak interactions, parity was not conserved. But it would take a dedicated experiment to prove it — after all, no one knew whether Θ and τ were the same particle or not — and that’s where Wu came in.
Wu decided to prepare a sample of cobalt-60, a radioactive isotope of cobalt which undergoes beta decay, transmuting into nickel. Wu’s idea was brilliant, because she realized that cobalt nuclei have a spin, and that by leveraging two separate techniques together, she could make all of the spins line up. First, she cooled the cobalt down to very low, cryogenic temperatures, which reduces their thermal vibrations down to negligible amounts. Then, while still at those ultra-low temperatures, she applied a large, constant, uniform magnetic field to them.
Normally, its collisions, vibrations, and other thermal effects that cause the spins of atomic nuclei to be randomized. So the low temperatures prevent that from occurring, while the large magnetic field causes the spins of every nucleus to all line up.
Why is that important?
You can illustrate this with your left hand. Point your thumb up, and curl your fingers. Note that if you look down at your thumb, your fingers appear to point in the clockwise direction. If the particle then decays, that “spin axis” (your thumb) still points up, and that should be imprinted on the new particles that emerge from the decay. This is fundamentally different than if you use your right hand, which is the mirror-image of your left hand.
If parity is conserved, then particles should behave “left-handed” and “right-handed” equally, with no preference for one or the other. After all, parity symmetry means mirror symmetry, and anything that a left-handed particle would do in this world, the right-handed version would do in the mirror.
What Lee and Yang predicted, if parity was violated, was that the decay would be asymmetric: the decay products of cobalt-60 would preferentially occur in a way that cared about which direction their spin axes were aligned, and that nature would exhibit a fundamental asymmetry. On the other hand, if they were wrong and parity were conserved, the decays would be symmetric, and aligning the spins in one direction would produce identical results to aligning them in the opposite direction.
In physics — and this is worth emphasizing — the only way you can find out how nature actually behaves is to perform a decisive experiment or to make a decisive set of observations. We only unlock the secrets of nature by asking the Universe questions about itself. No matter how certain the predictions of your theoretical calculations, you must confront your ideas and hypotheses with data obtained from the real world.
Wu’s experiment was a success, which is to say that she was able to detect whether the particles were emitted asymmetrically (and parity is violated) or symmetrically (and parity is conserved). To the surprise of many, not only did she establish that parity is violated, but that it’s violated by pretty much the maximal amount: almost 100% of particles displayed a preference for being emitted along the spin axis of the original cobalt-60 nucleus. Going back to the original question about Θ and τ, they were in fact determined to be the same particle: today it’s known as the kaon.
In many ways, this discovery marked the beginning of what would eventually grow into today’s Standard Model of elementary particle physics. The work was so important that the 1957 Nobel Prize for Physics was awarded for the investigation of parity laws which led to important discoveries regarding elementary particles. The prize, which is limited to three people, was awarded jointly to Chen Ning Yang and Tsung-Dao Lee, with no mention of Wu at all. In fact, Wu’s role in the discovery of parity violation — she was literally the one who experimentally determined it — was not publicly honored by any major professional organization until the Wolf Prize was founded in 1978, which was specifically designed to be awarded to living scientists and artists who were deserving of, but had not received, the Nobel Prize.
After her historical work on parity violation, Wu continued a remarkable career. She experimentally confirmed the Conserved Vector Current hypothesis; she proved that the charge conjugation © symmetry was also violated in the weak interactions; she was the first to experimentally obtain results from experiments involving entangled photons as related to the Einstein-Podolsky-Rosen paradox.
Wu also was an activist against sexism in physics, only obtaining equal pay to her male counterparts in 1975 and often was mis-addressed as “Professor Yuan,” her husband’s name, which she always corrected by informing them that she was Professor Wu. At one point, in a symposium at MIT, she asked the audience, “I wonder whether the tiny atoms and nuclei, or the mathematical symbols, or the DNA molecules, have any preference for either masculine or feminine treatment?”
Had Wu been justly awarded the Nobel Prize in 1957 along with Lee and Yang, she would have been only the second woman at the time to win the award, following Marie Curie. While it’s too late to right that wrong, we can now celebrate her life, her work, and her legacy every time we send a letter through the United States Postal Service. May we all wish a posthumous congratulations to Chien-Shiung Wu: the First Lady of Physics.
Starts With A Bang is written by Ethan Siegel, Ph.D., author of Beyond The Galaxy, and Treknology: The Science of Star Trek from Tricorders to Warp Drive.