Is The Universe Filled With Black Holes That Shouldn’t Exist?

Every object in the Universe is constrained by the laws of physics. Does this mean there are any black holes that shouldn’t exist?
When it comes to the objects that are found all throughout the Universe, most of them align with our theoretical expectations. Occasionally, however, scientists will find an object that seems to defy conventional wisdom. When this occurs, however, it’s usually not because there’s a flaw with our understanding of the rules that govern the Universe, but because we’ve modeled certain physical processes or environments too simplistically.
For black holes, the overwhelming majority of them originate from a supernova explosion occurring in a massive star near the end of its life. Over time, black holes can grow by merging with other objects and accreting additional mass, and they can form from the mergers of other objects as well. Theoretically, some black holes shouldn’t exist, and yet we see them anyway. Here’s what it all means.
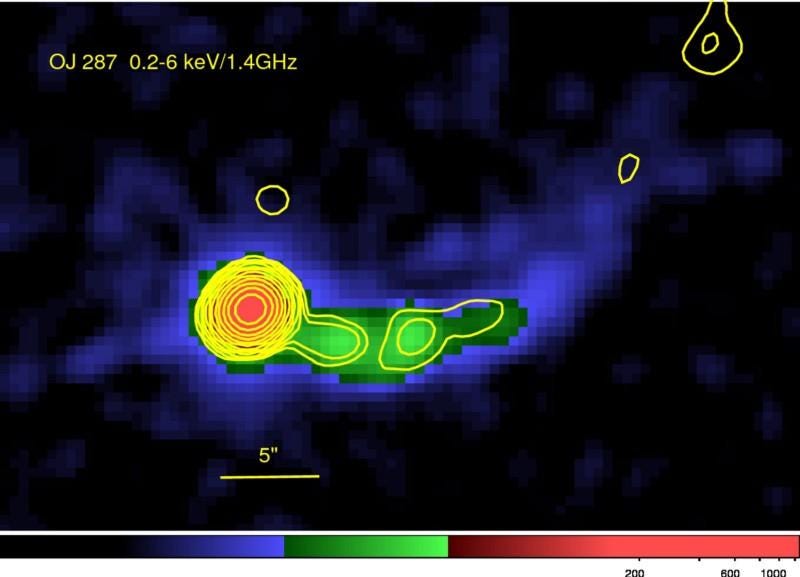
Whenever you try and make a prediction for what should exist in the Universe, you’re immediately constrained by the assumptions that you make. Conventionally, the story for how the Universe makes black holes is as follows:
- A cloud of molecular gas begins to collapse, fragmenting into small clumps that gravitationally grow larger and larger at a rapid rate.
- At some point, in the central regions of the clumps that grow large enough quickly enough, nuclear fusion ignites, marking the birth of a new star.
- The stars that are massive enough will burn through their core’s hydrogen, and then begin fusing helium into carbon, carbon into oxygen, and so on until the core contains iron, nickel and cobalt in the center.
- At this point, core fusion can occur no longer, and the interior of the star implodes, leading to a runaway supernova explosion for the outer layers.
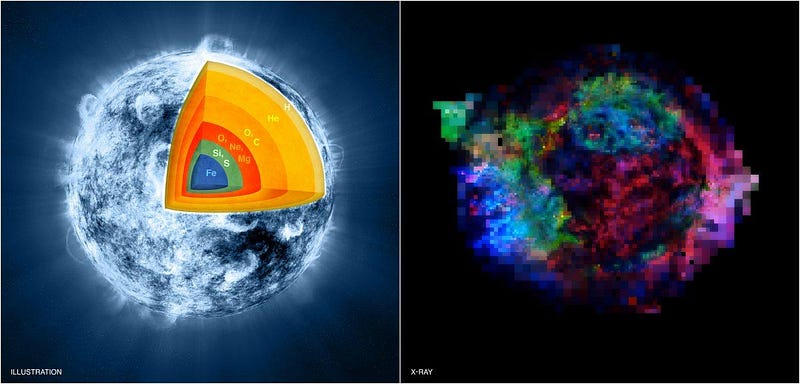
If your star is below a certain threshold, it produces a neutron star at the core; if it’s above that threshold, it produces a black hole. In theory, then, there should be a lower limit to the mass that a black hole in the Universe can have, and at lower masses, any other object should be identifiable as something other than a black hole.
In addition to that, stars are limited by how massive they can get and still remain stable as their lives progress. You cannot simply make more and more massive black holes by having more and more massive stars, and that’s because the more massive your star gets, the higher the core temperature of the star gets. At some point in a massive-enough star, your star’s temperature will cross a critical threshold: where the most energetic photons inside will spontaneously start producing electron-positron pairs.
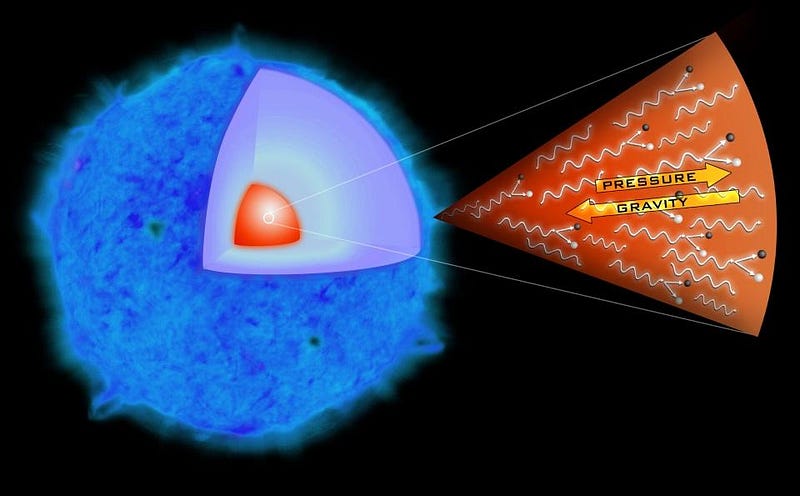
Whenever this occurs, the internal pressure drops, and the entire star is destroyed in what’s known as a pair-instability supernova. Therefore, you might reason, this should lead to a second region where black holes shouldn’t exist: above the threshold of the maximum mass black hole you can produce from a core-collapse supernova before the entire star tears itself apart.
And finally, there should be a supermassive limit as well: one where even if you produced a black hole very early in the Universe, and it grew by accretions and mergers at the maximum rate allowable by the known astrophysical processes at play in realistic environments, it could not have grown any larger. In theory, those are the three gaps we’d expect to find:
- a minimum mass to stellar-mass black holes,
- an intermediate gap at the high end of the stellar-mass range,
- and then a maximum mass for even supermassive black holes.
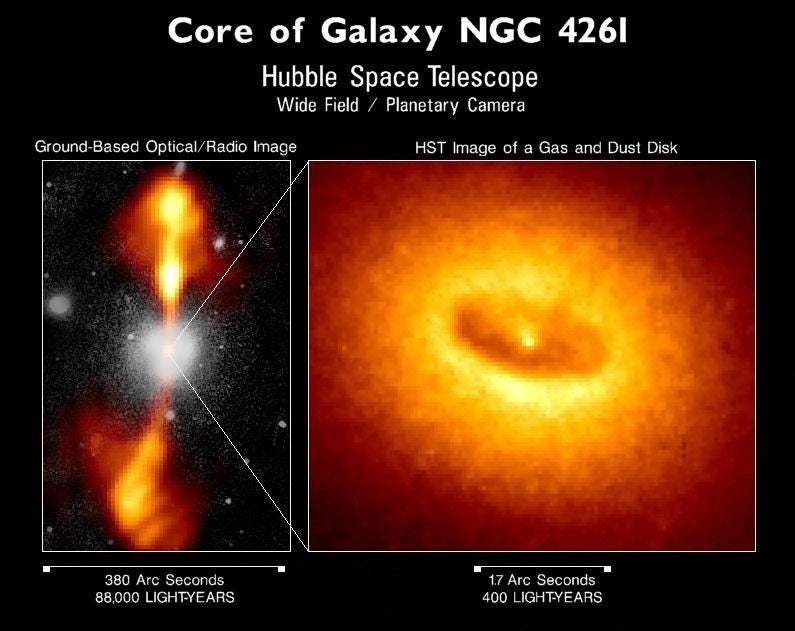
Of course, these anticipated “forbidden regions” are only forbidden based on certain assumptions that may or may not be correct, and it’s easy to assume all our assumptions are correct when these expectations line up with what we’ve seen so far.
But it’s important to remember that we have only a tiny fraction of the data we can hope to collect about black holes, and that most of the evidence for them is indirect: through X-ray emission data from gas near the central region of a system where a black hole is suspected to be. These mass estimates are not as robustly reliable as either the direct tracing of orbits or direct mass measurements from gravitational waves; they are often off by up to 50% where multiple measurements are available.
And since the advent of gravitational wave detectors like LIGO and Virgo, the game has really changed.
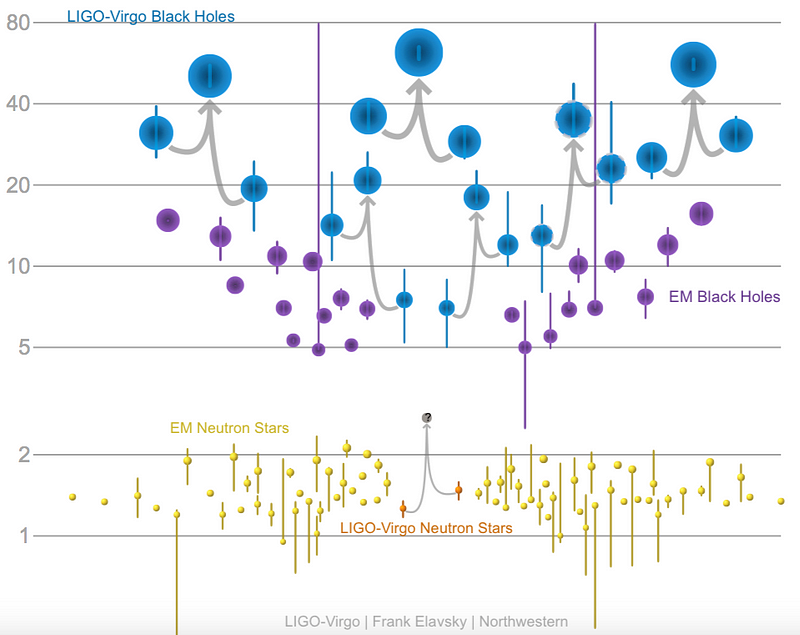
For one, that low-end gap, between neutron stars and black holes, is beginning to get filled in. There are theoretical limits to how massive any collection of particles can be before the force of gravity overcomes their ability to remain stable. For normal atoms, the Chandrasekhar mass limit (about 1.4 times the mass of our Sun) teaches us the upper limit of a white dwarf star, while for neutrons, the Tolman-Oppenheimer-Volkoff limit (about 2.3 times our Sun’s mass) gives the limit of a neutron star. If these bodies rotate, those figures can be increased by a small amount.
Meanwhile, X-ray binary measurements had never revealed a black hole below about 5 solar masses.
What lies between the most massive neutron star and a 5 solar mass black hole?
The answer is sure to be “black holes,” and the only real question is “how frequently?”
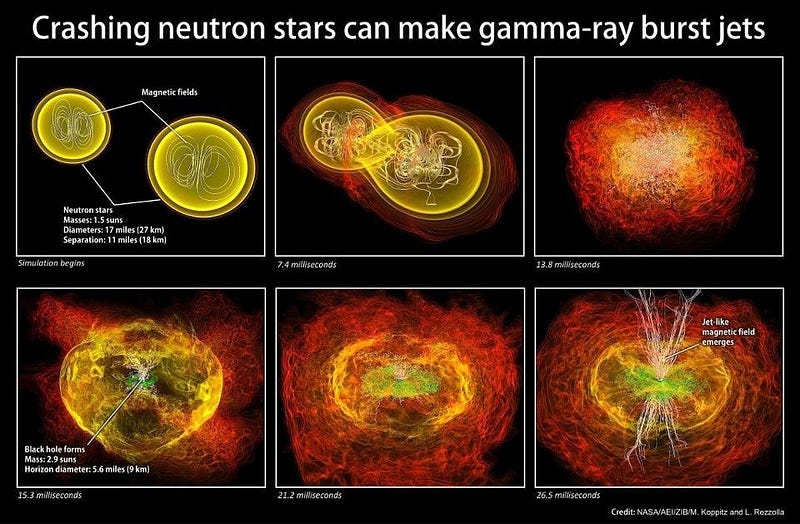
In 2017, astronomers witnessed — in both gravitational waves and from electromagnetic radiation — two neutron stars merging in what’s become known as a kilonova event. The gravitational radiation clearly revealed two neutron stars locked in a death-spiral, merging to become an object that falls right in that critical “gap” range. With just a little under 3 solar masses, it appeared to remain a neutron star for a fraction of a second, before collapsing into a black hole itself.
Are the only black holes in this “gap” region formed by merging neutron stars? Or are black holes in this regime formed just as frequently as high-mass neutron stars or 5 solar mass black holes? As LIGO and Virgo and other gravitational wave detectors both become more sensitive and build up more statistics, they will reveal the answer to this question.
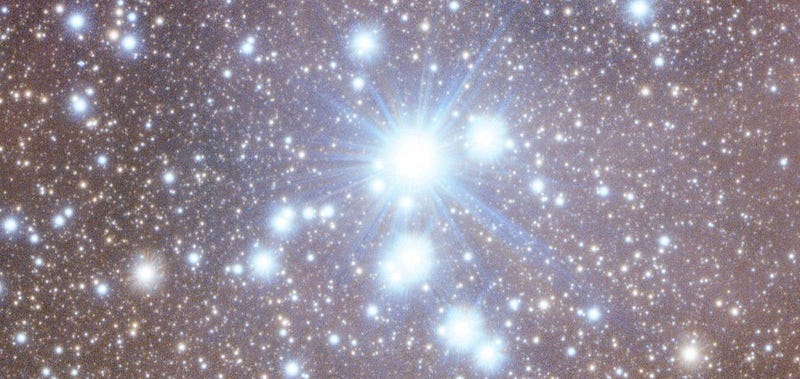
What about at the high end of the stellar mass range of black holes? It’s true that pair instability supernovae are real and are indeed a limiting factor, as they don’t produce black holes. However, there’s an entirely separate way to produce black holes that is not particularly well understood at this time: direct collapse.
Whenever you have a large enough collection of mass, whether it’s in the form of a cloud of gas or a star or anywhere in between, there’s a chance that it can form a black hole directly: collapse due to insufficient pressure to hold it up against gravitation. For many years, simulations predicted that black holes should spontaneously arise through this process, but observations failed to see a confirmation. Then, a few years ago, one came in an unlikely place, as the Hubble Space Telescope saw a 25 solar mass star simply “disappear” without a supernova or other cataclysm. The only explanation? Direct collapse.
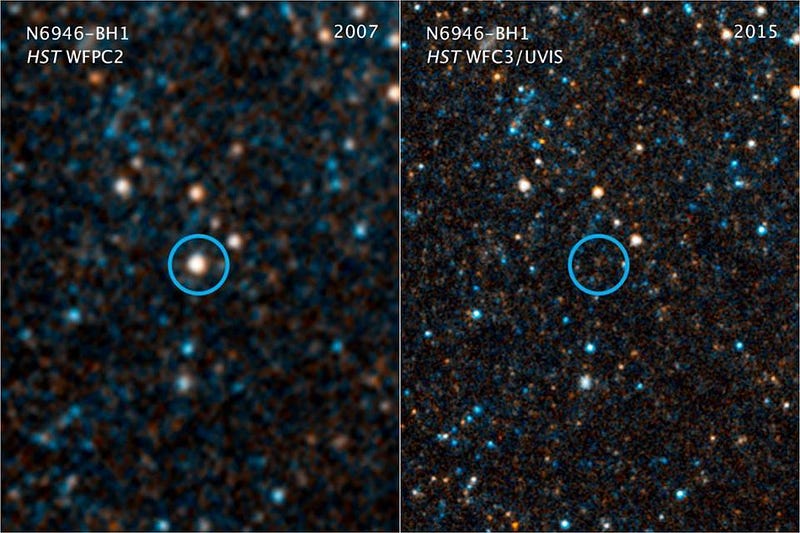
From the LIGO/Virgo data we’ve already collected, we know that it should be sensitive to black holes in the 50-to-150 solar mass range, and we haven’t seen any black holes inspiral and merge in that range at all. Scientists have concluded, based on these observations, that 99% of the stellar mass black holes out there must be 43 solar masses or below, and that this reinforced the theoretical idea of a mass “cliff” at about 50 solar masses.
But the definitive data is still to come, and this is actually a hotly debated area of study at present. Many scientists have noted that different metallicities (the abundance of heavier elements) can change the outcome of a star’s life cycle, and have reasoned that with the right values, those heavier black holes could be quite common. In addition, direct collapse makes these heavier black holes a real possibility.
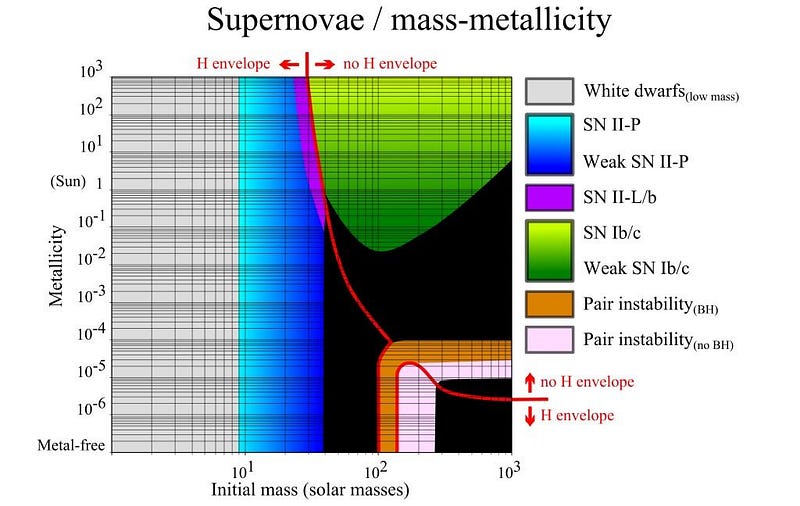
Finally, gravitational growth or accretions/mergers could lead to a quite substantial population in this mass range, especially if binary/trinary/quaternary/etc. systems of initially massive stars are abundant. Black hole mergers could be common and could occur sequentially (where a previously-merged black hole merges again), or black holes could consume substantial amounts of matter, and either mechanism could fill in this theoretical gap quite effectively.
It’s a very easy scientific mistake to make: to assume a simple scenario when your data doesn’t demand anything more complex, even if there’s relevant physics that’s sure to matter and alter the expected outcome. There’s an old saying that when your predictions match the data, you stop searching for possible errors, omissions, or oversimplifications. Yet as soon as we do that, we can easily mislead ourselves.
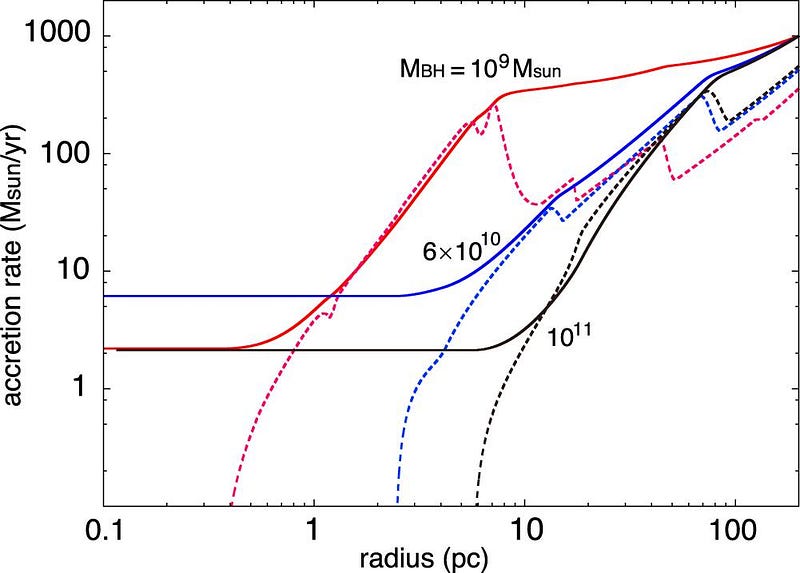
At the very high end, however, there truly is a limit. No matter how quickly you form black holes or how quickly they grow, there are physical constraints that limit how big a black hole can truly be after 13.8 billion years of cosmic history. As astronomers Kohei Inayoshi and Zoltan Haiman showed back in 2016, that mass limit caps out at around 60 billion solar masses. That appears to check out, as their estimates and our current suite of observational evidence line up incredibly well.
But if our Universe has taught us anything, it’s that the simplified assumptions we make about how the myriad of objects in our Universe behave are often oversimplified. What we currently perceive as the limits of black holes are sure to be widened in future years, as gravitational wave science continues to improve and reveal new truths about the Universe. Expect many bizarre headlines as we discover black holes “that shouldn’t exist,” because what we’re really discovering is just how astray a naive theoretical bias can lead us.
Ethan Siegel is the author of Beyond the Galaxy and Treknology. You can pre-order his third book, currently in development: the Encyclopaedia Cosmologica.