IceCube finds neutrinos from 47 million light-years away
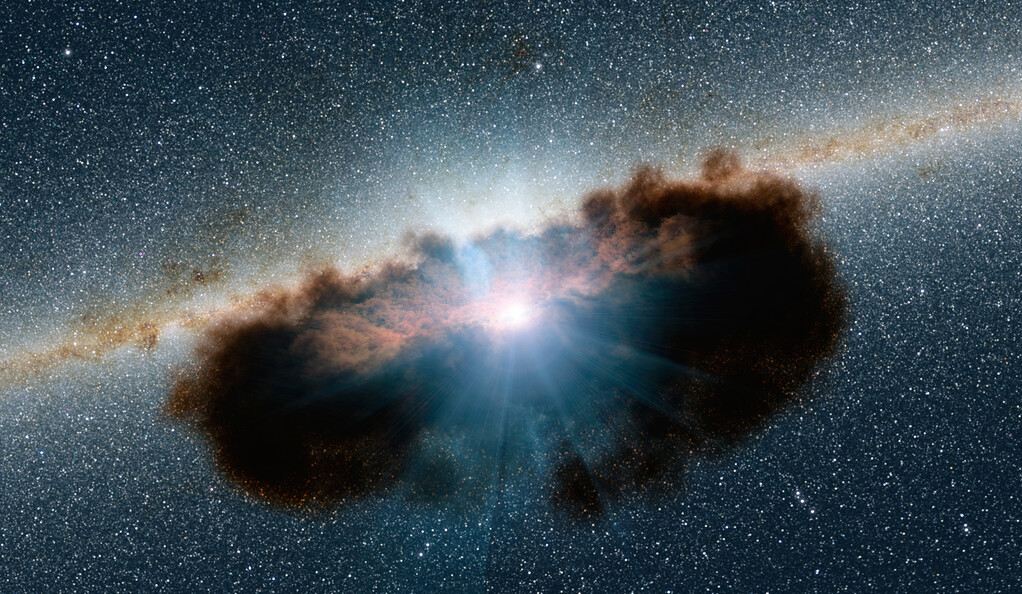
- Throughout the 20th century, only four known sources generated neutrinos: the Sun, Earth’s atmosphere, radioactive decays, and a nearby supernova back in 1987.
- However, neutrino observatories have advanced tremendously in the 21st century, led by IceCube: the world’s most sensitive detector, found at the south pole.
- With 10 years of cumulative observations, one nearby galaxy now stands out: Messier 77. It’s now been seen not only in light, but, with 79 excess events, in neutrinos as well.
Neutrinos are, in many ways, the most difficult species of known particle to detect at all. Produced wherever nuclear reactions or radioactive decays occur, you’d have to make a lead barrier that was approximately a light-year thick to have a 50/50 shot of stopping a neutrino in motion. Although there are many places neutrinos are made — in the Big Bang, in distant stars, in stellar cataclysms, etc. — the overwhelming majority of neutrinos we see come from just three sources: radioactive decays, the Sun, and from cosmic ray showers produced in Earth’s upper atmosphere.
Still, the IceCube neutrino observatory, located deep under the ice at the South Pole, has revolutionized the science of neutrino astronomy. Since 2010, it’s sensitive to neutrino interactions within more than one cubic kilometer of glacial ice, allowing us to detect neutrinos from all across the Universe, including from active galaxies whose jets point right at us: blazars. Now, in a neutrino first, it’s detected 79 excess events coming from a nearby, dust-obscured active galaxy: Messier 77. This galaxy, just 47 million light-years away, is the first in the nearby Universe to be detected via its unique neutrino signature, taking astronomy into new, uncharted territory.
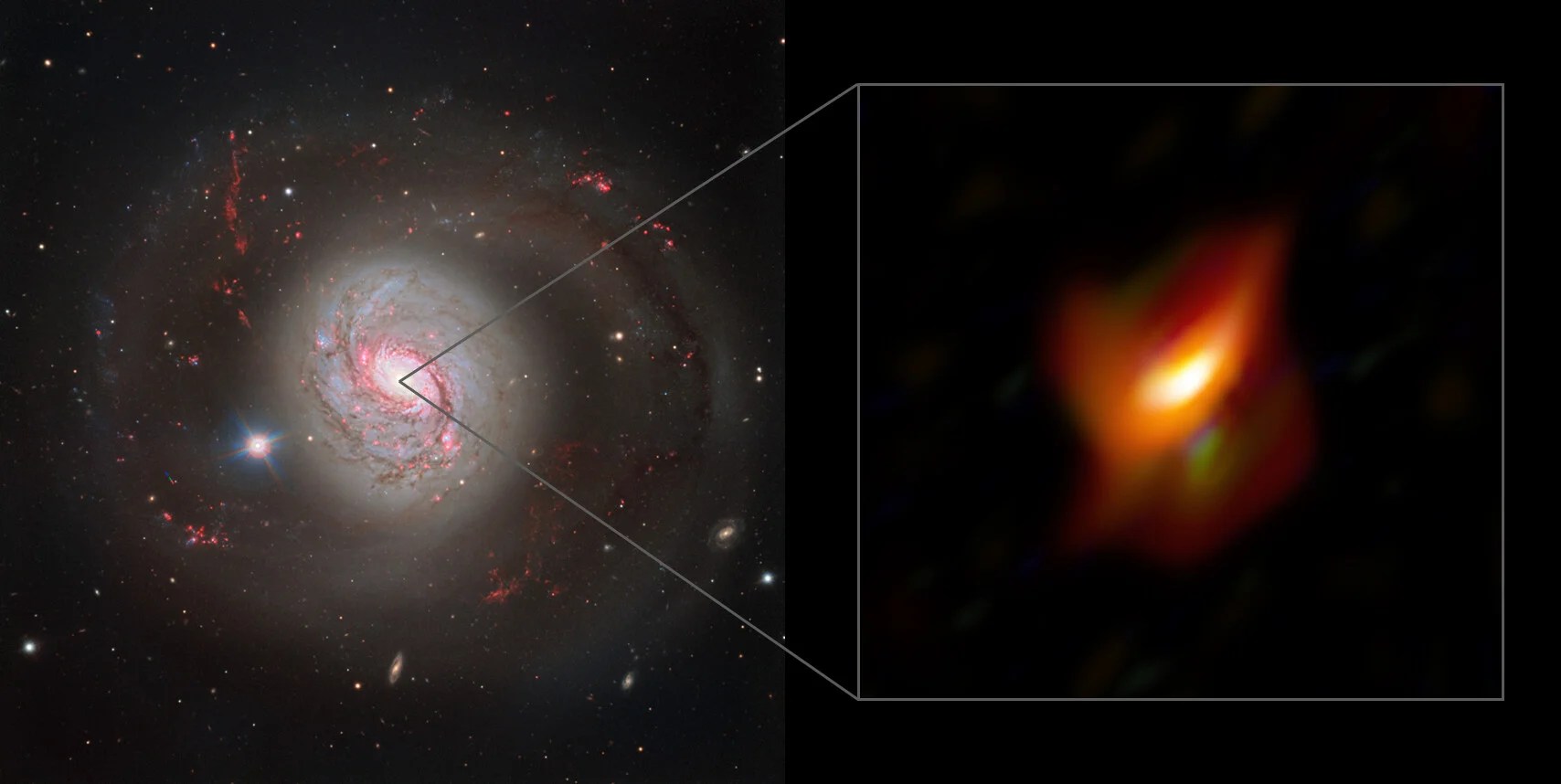
In theory, there’s more to the Universe than just the light we observe. There’s an entire high-energy Universe, filled with astrophysical objects — some large, some small; some very massive, some more modest; some extremely dense, others more diffuse — that can accelerate matter of all types to extraordinary conditions. They can produce not only high-energy light, such as X-rays and gamma rays, but also particles and antiparticles of all varieties: protons, nuclei, electrons, positrons, as well as unstable particles that are destined to decay.
Many nuclear processes, including fusion and fission reactions, as well as a wide variety of decays, will produce neutrinos and antineutrinos as part of their particle content. This is extremely interesting from an astrophysical perspective, as the very fact that neutrinos have such a tiny interaction cross-section with normal matter means they can largely travel through the Universe, even through dense, matter-rich environments, in a practically unstoppable fashion. Other than the fact that the neutrino flux spreads out as we move farther and farther away from the source, the neutrinos (and antineutrinos) that impact the Earth are very similar to what we’d expect to see if there were no interfering matter along the way at all.
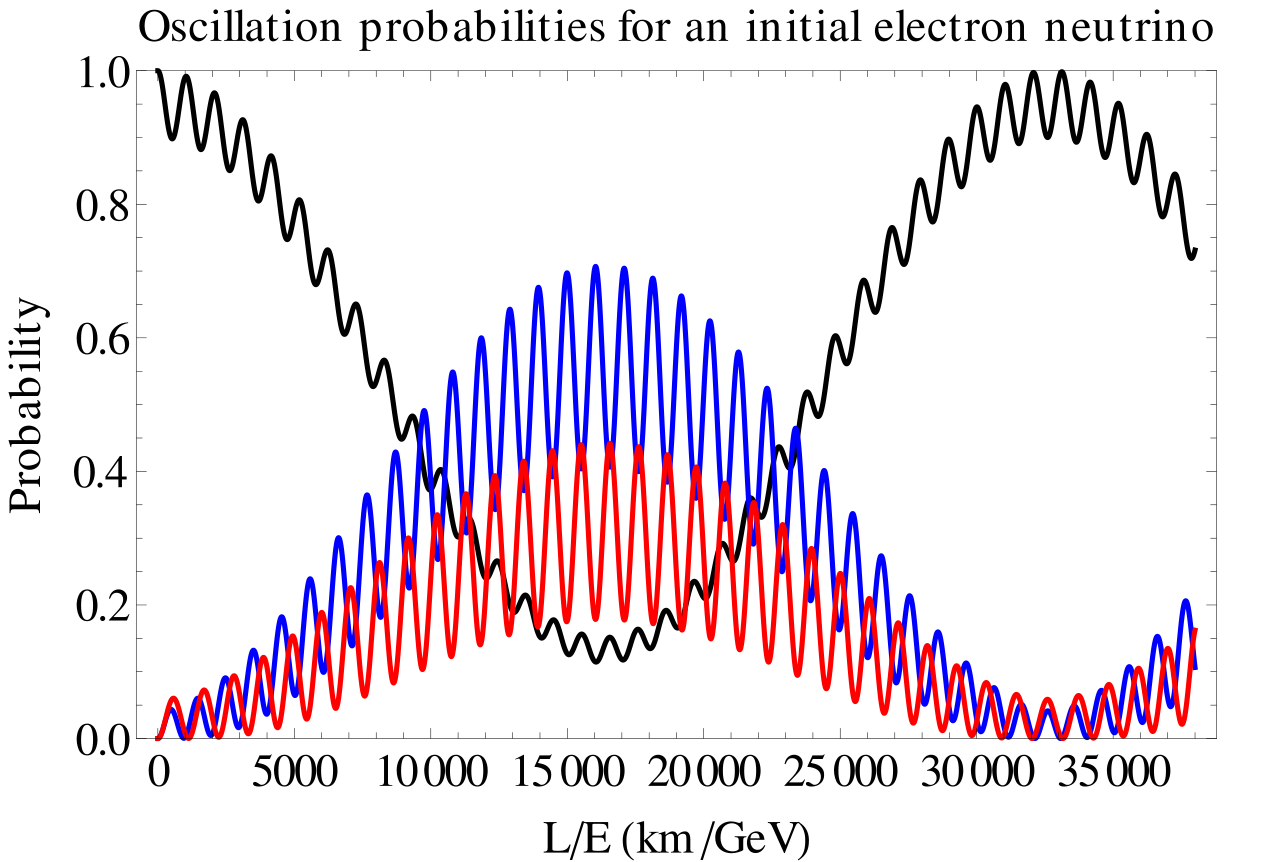
The matter that the neutrinos (and antineutrinos) do pass through, in fact, play only one major role: they can alter what sort of “flavor” of neutrino one observes in a detector. There are three different types of neutrinos that we can measure: electron, muon, and tau neutrinos. Whenever neutrinos are first made, the specific flavor of neutrino that is required to conserve a specific quantum number — lepton family number — is the one that’s produced.
However, as neutrinos travel through the Universe, they interact with other quanta, both real and virtual. Through those interactions, they can oscillate from one species into another. Therefore, when they arrive at your detector, the “flavor” of neutrino that arrives may be different from the flavor that was first created. That’s why, ideally, you’d build neutrino detectors that are sensitive to all three of the possible flavors, and moreover, can distinguish between them.
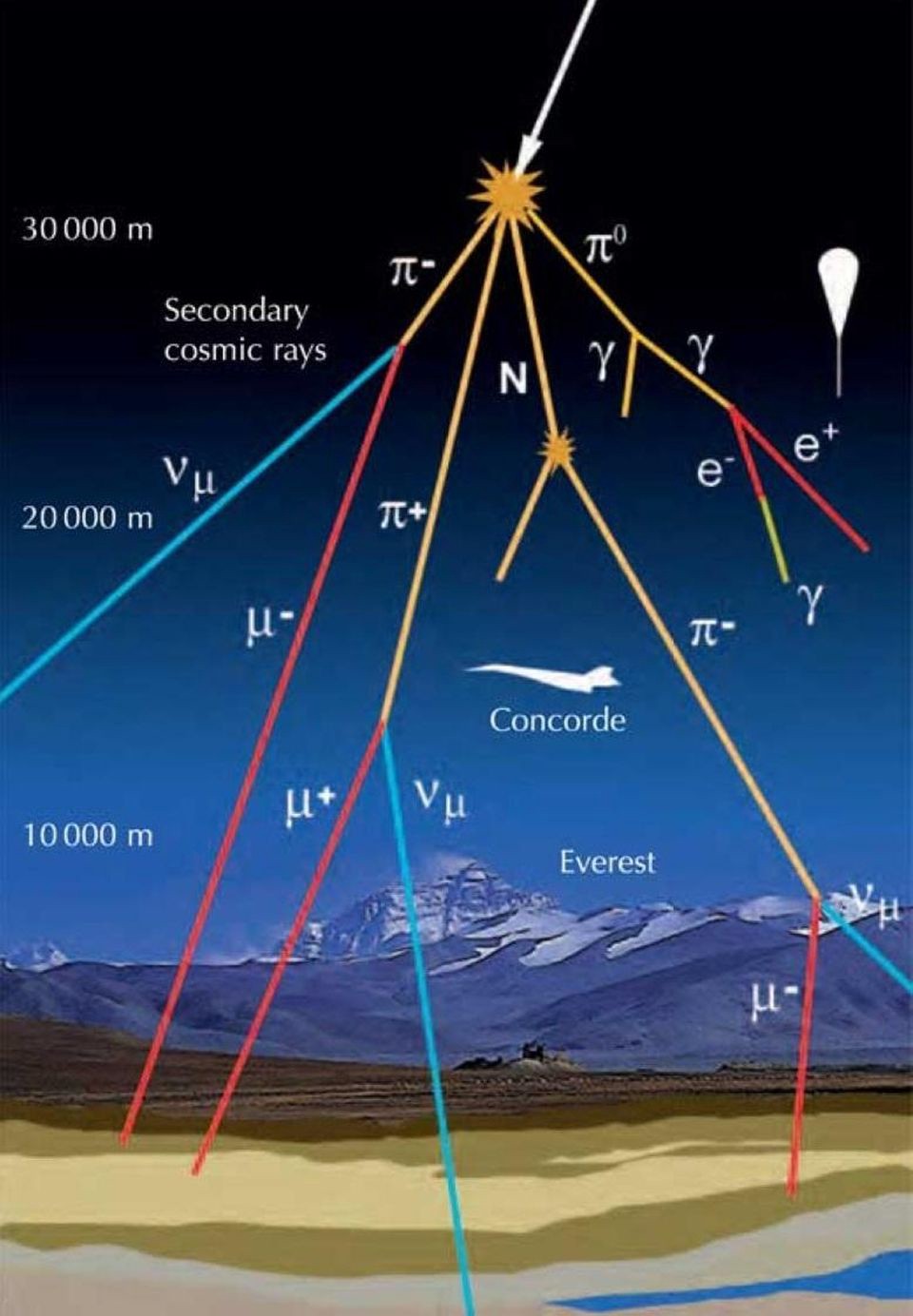
The original neutrino detectors that we built were sensitive only to the electron flavor of neutrino: the only one we initially knew about. When we began measuring neutrinos from the one nearby source we were certain would be creating them, the Sun, we noticed immediately that we were only detecting about a third of the total neutrinos that we predicted should’ve been there.
This solar neutrino deficit was only resolved decades later, when we combined large data sets from solar neutrino experiments, from reactor and beamline neutrino observations, and from atmospheric neutrino experiments — that is, experiments that measured the neutrinos that arise from high-energy cosmic rays striking Earth’s atmosphere — all pointed toward the same conclusion. These neutrinos came in three varieties, were all massive, and whenever a measurement or interaction with another quantum particle took place, must always take on one of those three flavors: electron, muon, and tau.
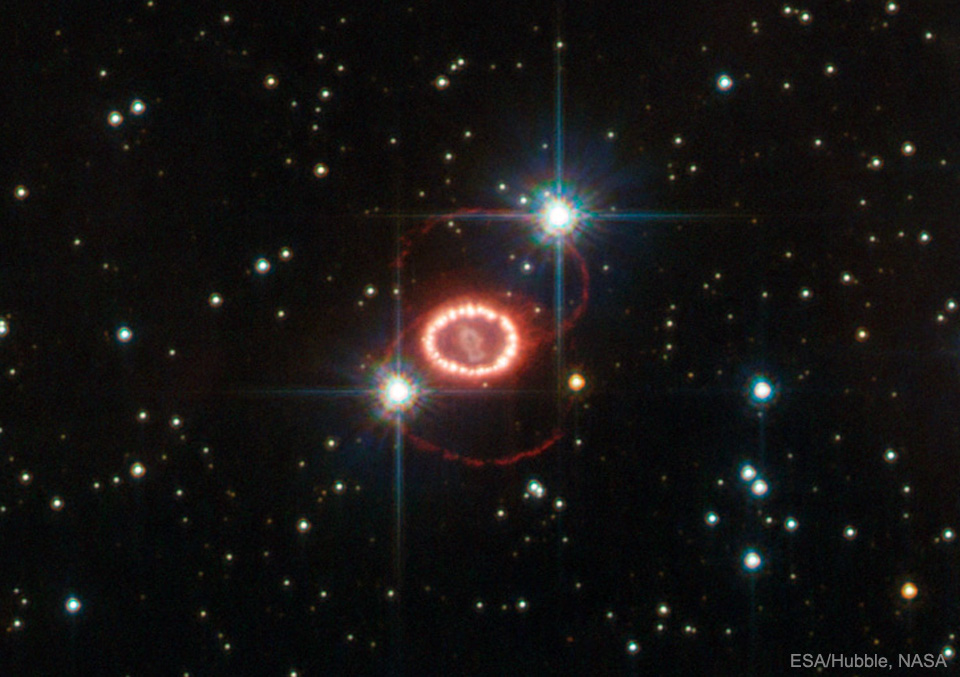
In fact, the only exceptions to those types of neutrinos that we saw:
- neutrinos created in the Sun,
- neutrinos created by a laboratory reaction, like a particle accelerator or a nuclear reactor,
- and neutrinos created in Earth’s atmosphere, arising from cosmic ray showers,
came from high-energy astrophysical cataclysms themselves. The first one was seen in 1987, when the light from a supernova arrived from just 165,000 light-years away: in a satellite galaxy of our own known as the Large Magellanic Cloud.
Although there were only about ~20 neutrinos arriving across three separate detectors, they were coincident in time, energy, and direction with the neutrinos produced from a core-collapse supernova reaction. We quickly realized that neutrino-creating reactions were occurring all over the Universe, and that we could detect them with sufficiently large volumes of material for them to collide with, and sufficiently sensitive detectors surrounding them in terms of momentum and energy resolution. That was part of the motivation for building the most sensitive neutrino detector on Earth: IceCube.
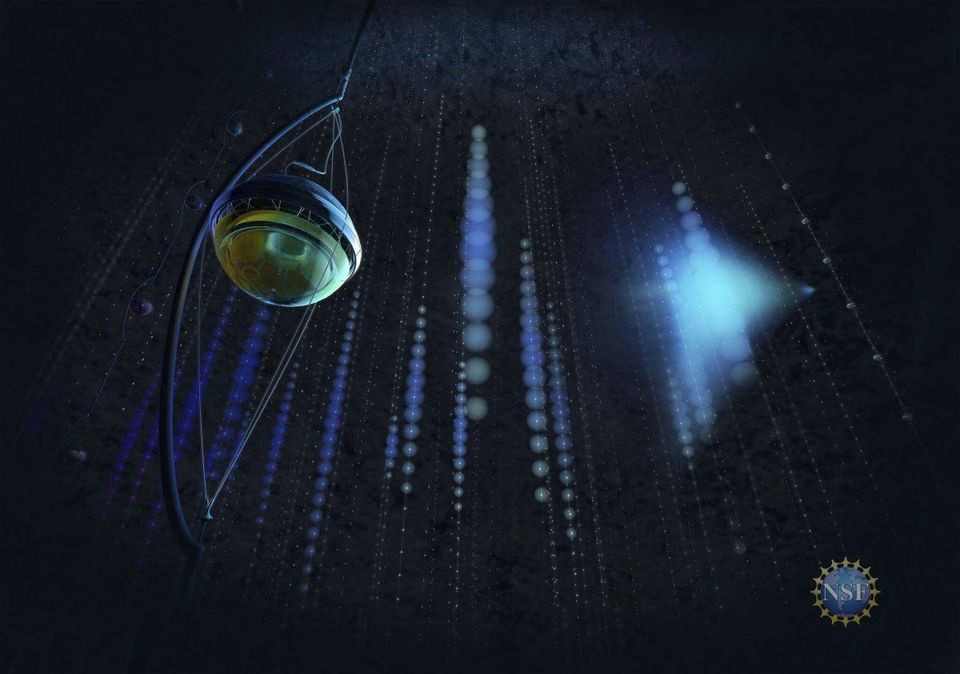
Made up of 86 string detectors that descend into a cubic kilometer of ice at the South Pole, IceCube became fully operational more than a decade ago: back in May of 2011. When neutrinos — from any source — strike the glacial ice, they produce secondary particles of all varieties, so long as there’s enough energy to create them via E = mc². Although all of these particles must travel either at (if they’re massless) or below (if they’re massive) the speed of light, that restriction applies to the speed of light in a vacuum: i.e., in empty space.
But because these particles are traveling through ice, not the vacuum of empty space, they can, and often do, travel faster than light in this particular medium, where the speed of light is only about ¾ths of its vacuum value. If a particle gets created moving at more than about 76% of the speed of light in vacuum, it will interact with the (ice) particles around it, emitting a mix of blue and ultraviolet light in a conical shape, the characteristic signal of Cherenkov radiation. By reconstructing the various Cherenkov radiation signals, we can reconstruct specifically where and at what energies these particles were created with, enabling us to reconstruct the neutrino events that triggered them.
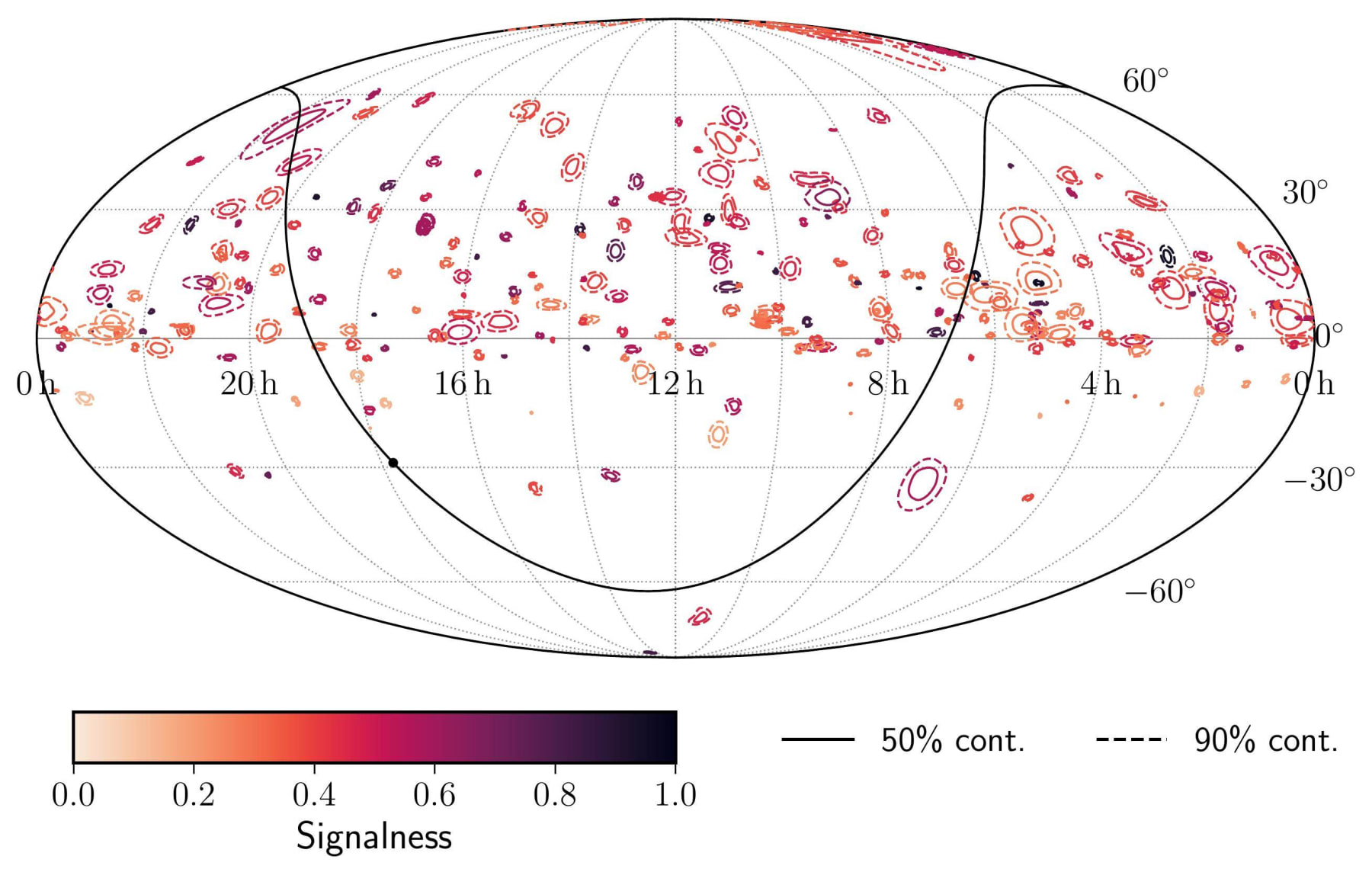
Since 2011, when the full detector became operational, certain astrophysical signals that had never been identified via their neutrino signatures before suddenly came into view of IceCube. The most spectacular such signal came from gamma-ray flaring blazars: TXS 0506+056, most famously. A blazar lies at the heart of an active galaxy, where the galactic nucleus consists of an actively feeding supermassive black hole. Normally, these black holes produce jets of collimated, high-energy radiation that are emitted perpendicular to the accretion disk around the black hole. But in the case of a blazar, that jet points directly at us.
Since that first detection, two other such blazars were seen in neutrinos by IceCube as well: PKS 1424+240 and GB6 J1542+6129. Although their neutrino signatures were less powerful and robust than the first blazar detected by IceCube, they still stood out above the diffuse neutrino background also seen by IceCube. All you ever need, if you want to identify a physical source for a signal that you’re seeing, is a signal that stands out above the noise background (and other backgrounds) of your experiment. The fact that we also have a gamma-ray map of the sky, as well as other wavelengths, helped us identify these sources as the origins of these high-energy neutrinos.
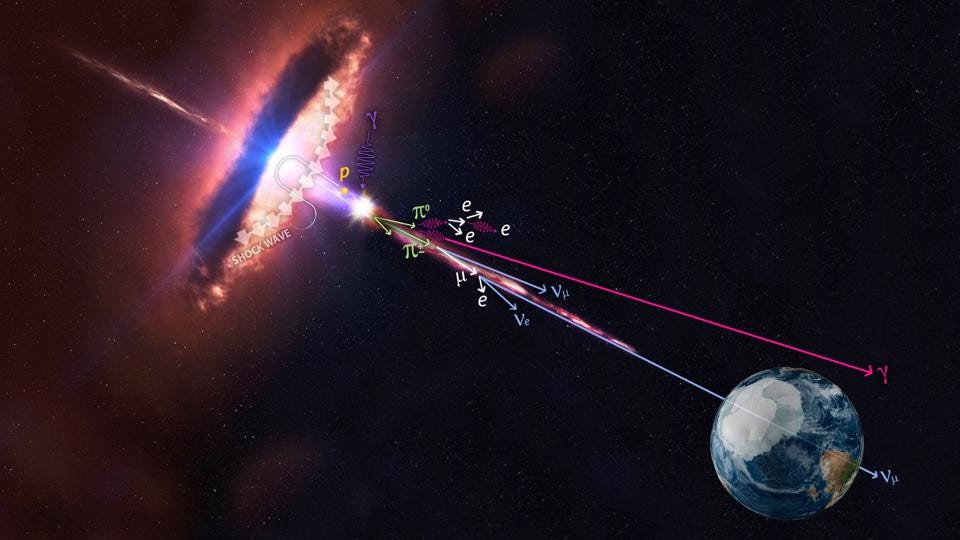
Even from billions of light-years away, some of these blazars gave off neutrino signatures that stood out spectacularly. But in between the very, very near and the very, very far, there was a tremendous gap. It was hoped by many that IceCube would be sensitive to supernova-produced neutrinos, but the only suspicious signal ever seen was shown to just be a coincidence. IceCube would indeed be capable of spotting neutrinos produced via a core-collapse supernova, but it would have to be very close by: closer than any supernova that’s occurred since 2011.
However, there were a great number of high-energy neutrino candidate events seen by IceCube: known as “alert events,” as they offered the possibility of being astrophysical neutrino sources, rather than a background event produced in Earth’s atmosphere. One strategy has been to attempt to correlate these events with possible high-energy sources in the sky: either known sources of high-energy light, of supermassive black holes, or of high-energy cosmic ray particles, which themselves might correlate with supermassive black holes as well. These observations have placed the tightest constraints to date on the abundance of astrophysical neutrino sources all across the Universe.
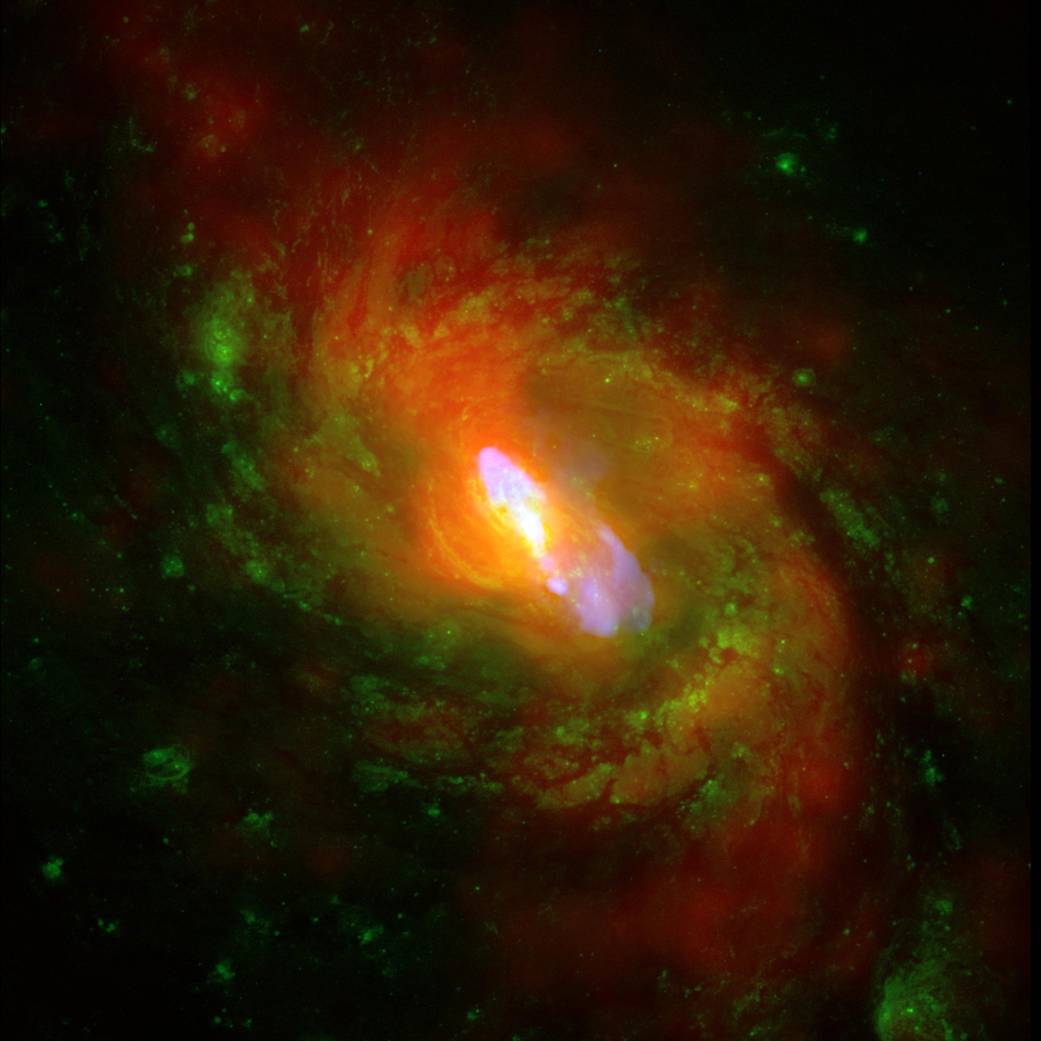
But in a landmark new study, the IceCube collaboration did see something that surprised many: an “intermediate” source of astrophysical neutrinos, one arising from a relatively nearby galaxy just 47 million light-years away. The galaxy Messier 77 — also known as NGC 1068 — has a number of features that makes it extremely interesting to astronomers.
- It’s a “double spiral” galaxy, with a diffuse outer spiral surrounding the main spiral: evidence of a recent gravitational interaction.
- It has a dusty nuclear region, about 12 light-years across, that emits an intense radio jet and strong emission lines.
- It’s also emitting X-rays from that core: the very central region.
In fact, all of these facts indicate activity from the central black hole, making this a galaxy with an active galactic nucleus. In fact, this galaxy was the very first of an entire class of active galaxies known as Seyfert galaxies, as astronomer Carl Seyfert first identified this class with Messier 77 as the archetype. Messier 77 has a supermassive black hole that’s about four times as massive as the Milky Way’s; it’s about 170,000 light-years in diameter; and despite its appearance, it isn’t face-on as you might think, but is inclined to our line-of-sight at about 40 degrees. It recedes from us at ~1,100 km/s, caught up in the expansion of the Universe.
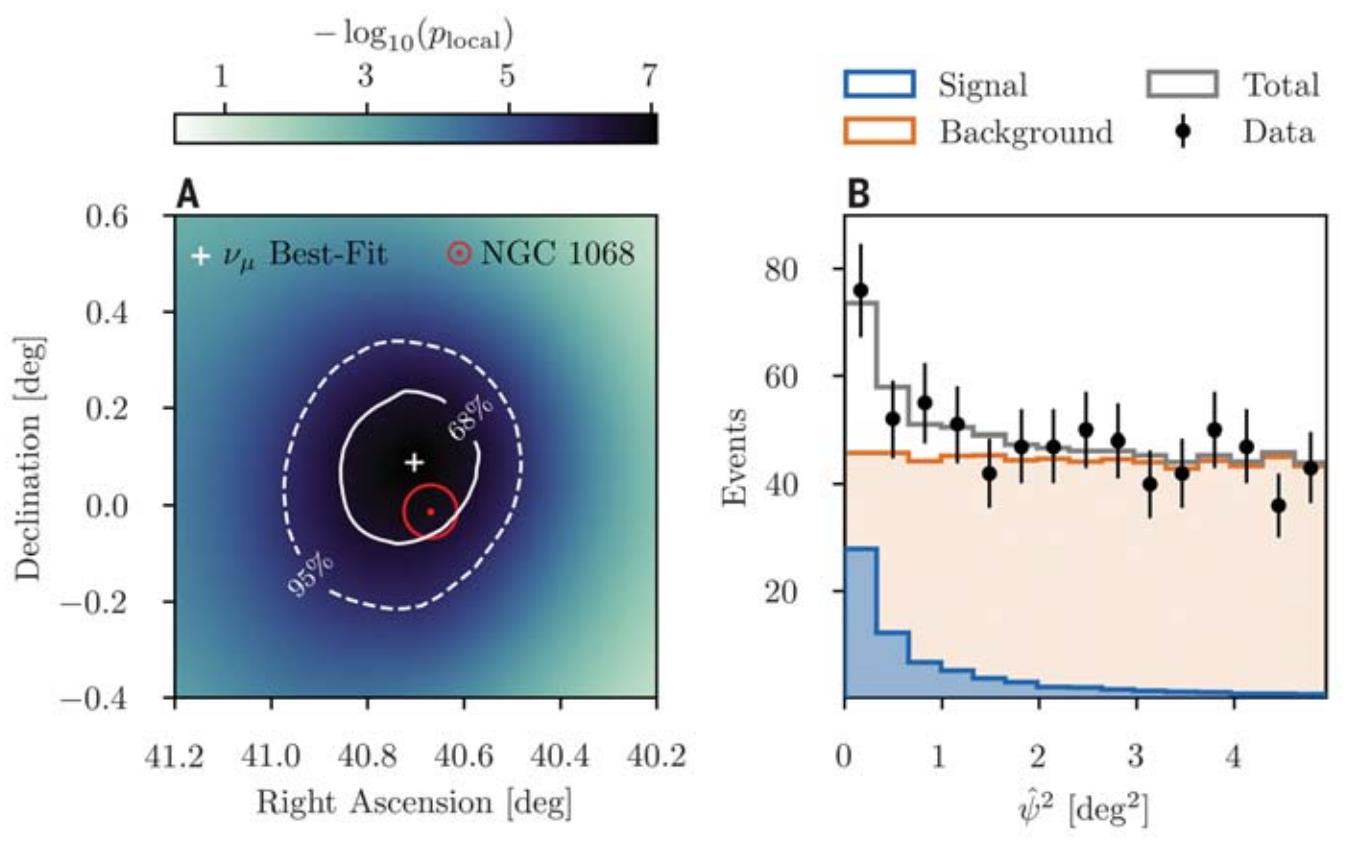
But now there’s a new reason to be interested in Messier 77: it’s now been identified, thanks to IceCube, as an extragalactic neutrino source! It was the most significant location of muon neutrinos observed above both the diffuse background and outside of the other known extragalactic neutrino sources. With 79 excess neutrinos at high energies (more than one trillion electron-volts) detected over the atmospheric and diffuse astrophysical neutrino background, it can now be claimed that we are, in fact, seeing neutrinos — regularly and over time periods of multiple years — arising from a nearby active galaxy.
Moreover, the IceCube team, for the very first time, was able to estimate the neutrino flux coming from a Seyfert galaxy such as this: about 16 muon neutrinos, per TeV (tera-electron-volt) per square meter per year, coming from this source. Most of the neutrinos that arrived were in the energy range of 1.5 TeV to 15 TeV, perhaps indicating the peak of neutrino energy production in this astrophysical environment. If we assume that this galaxy is, in fact, 47 million light-years away and that the other two flavors of neutrinos come in equal quantities, we can use that data to make the first-ever estimate of how much energy is emitted from a dusty, active galaxy in the form of neutrinos.
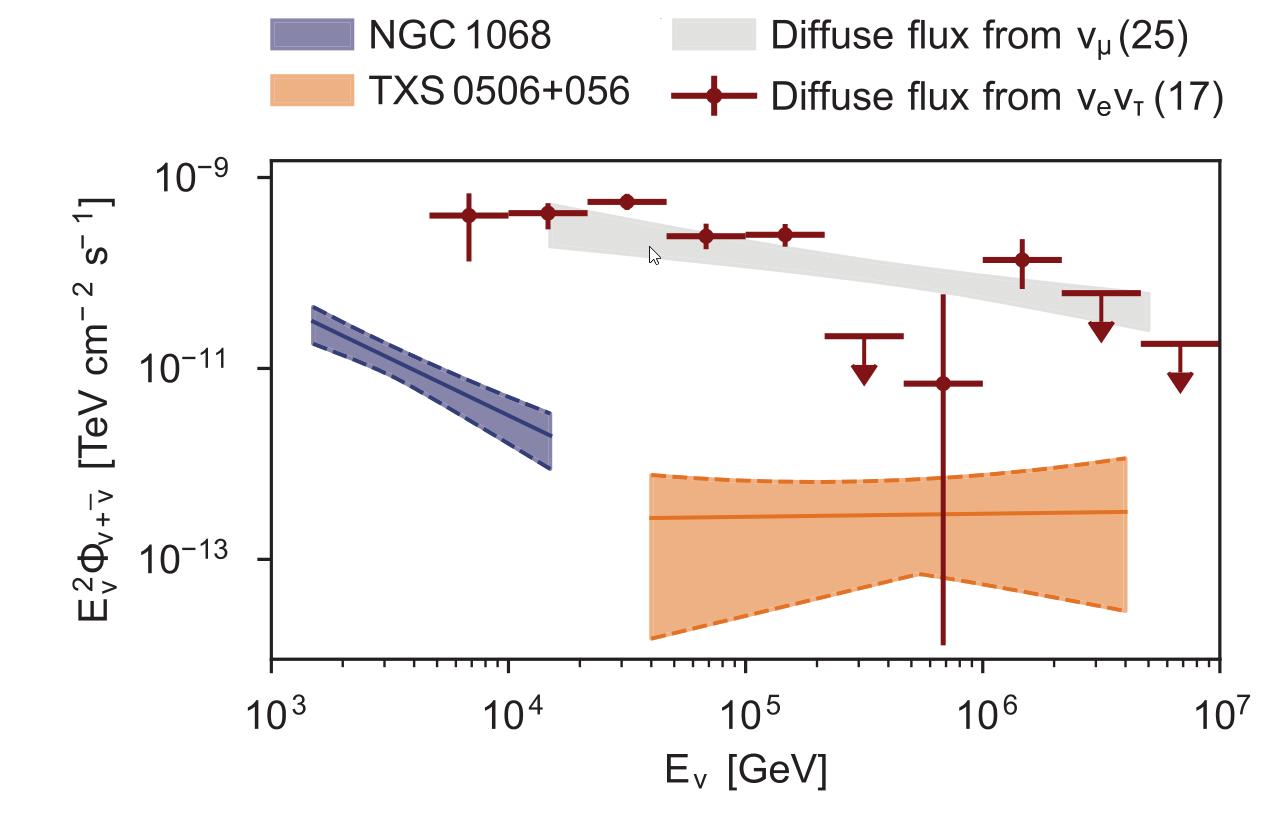
Remarkably, the number we get is about 750 million times the energy emitted by the Sun: all in the form of neutrinos, all from an active galaxy whose central supermassive black hole only weighs in at about 15 million times the Sun’s mass. For comparison, because this active galactic nucleus is also a gamma-ray emitting source, this is eighteen times as much energy in the form of neutrinos than is emitted in the form of gamma-rays. This may not be evidence of such a severe inherent difference, however; neutrinos don’t interact with the dusty surrounding medium, but gamma-rays do, providing a possible reason that the gamma-rays might be suppressed.
Perhaps even more excitingly, it tells us that we may want to look at another nearby Seyfert-type galaxy — NGC 4151, that’s just 52 million light-years away — as another possible extragalactic neutrino source. It tells us that, in the nearby Universe, there is at most one active neutrino-emitting active galactic nucleus similar to Messier 77 in every cubic box ~70 million light-years on a side. And, finally, it tells us that there are at least two populations of cosmic neutrino sources: from dusty active galaxies and from blazars, and they have different densities, energies, and luminosities to them. IceCube, at long last, is showing us what’s out there in the high-energy neutrino Universe. Combined with electromagnetic radiation, cosmic ray detectors, and gravitational wave observatories, the multi-messenger Universe is finally coming into focus.