How Do Cosmic Particles Break The Universe’s Energy Limit?
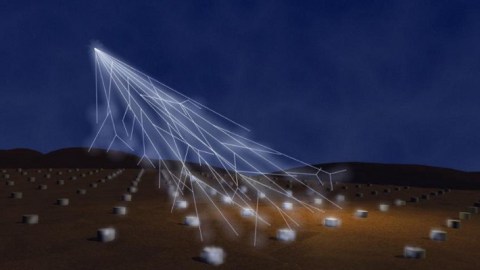
Cosmic rays aren’t just limited by the speed of light.
Even among non-scientists, it’s well-understood that there’s an ultimate speed limit to the Universe: the speed of light. If you’re a massless particle, like a photon, you have no choice but to move exactly at that speed as you travel through empty space, which is 299,792,458 m/s, or the speed of light in a vacuum. If you’re a massive particle, you can never reach that speed, but rather can only approach it. No matter how much energy you put into that particle, it will always move slower than light.
But that doesn’t mean that particles can move as close to the speed of light as they want, unimpeded. The Universe itself isn’t quite empty, as there are both massive particles and photons permeating all of space. At normal energies, they don’t play much of a role, but at very high energies, these particles exert a significant frictional effect, forcing those particles to slow down below a specific energy limit. At least, they ought to, but for almost 30 years we’ve been observationally finding particles that exceed this limit. Here’s the cosmic story behind what’s really going on.
The highest-energy particle we’ve ever produced on Earth is at CERN’s Large Hadron Collider. With energies reaching approximately 7 TeV, or around ~7000 times the rest-mass energy of the proton (from Einstein’s E = mc²), these particles move at 299,792,455 m/s, or 99.999999% the speed of light. This might seem fast, but protons with these energies are free to travel through the Universe without much worry.
What would a faster proton have to worry about?
Believe it or not, the answer is “the most common quantum of energy in the Universe,” which is the photon. Even though we think of photons as mostly coming from stars — which they do — those are only for the photons that have been created over the past ~13.7 billion years or so. Way back in the earliest stages of the Big Bang, far greater numbers of photons existed: more than one billion for every proton or neutron in the Universe. Today, those photons are still around, more diffuse and lower in energy than ever before. But we can not only detect them; we can figure out what their properties are.
Permeating every cubic centimeter of space, or approximately half the size of the last joint of your ring finger, there are 411 photons left over from the Big Bang in that volume. If you were to saw off half of your ring finger and leave it floating in space, more than ten trillion such photons would collide with it every second. Even though they’re exceedingly low in energy, with a mean energy of ~200 micro-electron-Volts, they’re the most abundant type of particle in the Universe.
In our own corner of the cosmic neighborhood, this number is absolutely dwarfed by the number of photons coming from our Sun, but that’s only because we’re so close to the Sun in space. While deep images of outer space reveal billions upon billions of stars clustered in trillions of galaxies within the observable Universe, the overwhelming majority of the Universe’s volume consists of intergalactic space. In those regions — which represent the places where cosmic particles spend the majority of their time traveling — it’s the leftover photons from the Big Bang that are the most common.
So what is it, then, that happens to particles as they travel through intergalactic space?
The same thing that happens to your hand when you stick it out of your car window as your vehicle travels down the highway. When your car is stationary, only the moving air molecules collide with you, and only at the low speeds/energies at which they travel relative to your stationary hand. When your car is in motion, however, your moving hand will preferentially collide with greater numbers of particles in the direction your hand is in motion. And the faster you go, the greater:
- the rate of collisions with air molecules,
- the force experienced by your hand,
- and the energy exchanged between the particles and your hand with each individual collision.
In fact, every time you double your vehicle’s speed, the force on your hand from collisions with air molecules quadruples.
For cosmic particles, the story is similar. For a stationary particle, it experiences an equal rate of equal-energy collisions from these leftover photons in all directions. If the particle isn’t stationary, but rather is slow-moving, the photons left over from the Big Bang collide with it from all directions relatively equally, but they’re more likely to collide in the direction the particle is moving. Additionally, there will be a slight energy shift: the collisions that occur head-on, between the particle and photons moving in the opposite direction, will impart more energy to the particle than photons striking it from any other direction.
However, at even the speeds achievable at the Large Hadron Collider, the effects of these photons can be neglected. Even for particles that travel through the intergalactic medium for billions of years, even at 99.999999% the speed of light, these common photons are so low in energy that they fail to slow down these particles by even a single meter-per-second, cumulatively, over the history of the Universe.
But at very, very high energies, things start to get interesting. The reason? Whenever two things collide, there are three options for what can occur, even though we normally only consider the first two.
- They can collide elastically, where the two objects scatter off of one another, exchanging energy and momentum but conserving both.
- They can collide inelastically, where the two objects conserve momentum but lose energy, wholly or partially sticking together in the process.
- Or they can collide and — if enough energy is available — create new particles (and antiparticles) through Einstein’s most famous equation: E = mc².
Colliding a photon with a fast-moving cosmic particle, like a proton (which most cosmic rays are observed to be), won’t have much of an effect if there’s not enough energy (in the center-of-momentum) for E = mc² to do anything interesting. But as the cosmic particle in question gets more and more energetic, eventually the quantum effects that arise from this third phenomenon start to become important.
At around one million times the energies that protons can achieve at the Large Hadron Collider, the fact that photons can “fluctuate” into a state where they behave as electron-positron pairs starts to matter. When protons reach an energy that exceeds about 10¹⁷ electron-Volts, here’s what occurs. In the center-of-momentum frame, the proton “sees” the photon as having about 1,000,000 electron-Volts of energy, boosted from its original ~200 micro-electron-Volts. This matters, because the electron and positron each have a rest-mass energy of around 500,000 electron-Volts; if you can create them, you can interact with them.
Once protons start colliding with these electrons (and positrons), they start losing energy far more rapidly. Each electron (or positron) collision drains about 0.1% of the original proton’s energy; even though these events are rare, they can add up over the millions of light-years that separate the galaxies from one another. However, this effect alone isn’t enough to cap the allowable energy for cosmic ray protons.
But there should be a cap: when the center-of-momentum energy rises high enough that a proton colliding with a photon has enough free energy, again via Einstein’s E = mc², to produce a subatomic particle known as a pion (π). This is a much more efficient energy-draining process, as each pion produced lowers the proton’s original energy by about 20%. After traveling for just ~100–200 million years through the intergalactic medium — a blip in time compared to the 13.8 billion year age of the Universe — all protons should fall below that limiting energy: around 5 × 10¹⁹ electron-Volts.
But ever since we first began measuring the energies of cosmic rays, we’ve discovered evidence for particles that exceed that maximum energy: the most extreme examples of ultra-high-energy cosmic rays. 30 years ago, the Fly’s Eye camera in Utah observed a cosmic particle with 3.2 × 10²⁰ electron-Volts of energy, and was immediately named the Oh-My-God particle. A follow-up detector, HiRes, confirmed the existence of multiple particles (about ~15 or so) exceeding this limiting energy threshold. And, currently, the Pierre Auger Observatory continues to detect a significant number of events that possess energies that are robustly above this theoretical maximum.
How is this possible? Before your mind goes to the most fantastic explanations imaginable, like “relativity is wrong,” consider these other options.
- These high-energy particles are produced close by, so they don’t have time to drop below the limit.
- The highest of these high-energy particles aren’t made of protons, but something else that’s heavier and has a higher energy limit.
- Or that active, supermassive black holes can accelerate protons to extreme energies — a cosmic Zevatron — and they remain above that limit by the time they reach us.
More modern observatories can pinpoint the directions from which these particles came, and determine that they’re not correlated with any particular set of directions on the sky. They’re not correlated with features within our own galaxy, nor neutron stars, nor active supermassive black holes, nor supernovae, nor any other identifiable features.
However, there is some fairly good evidence that at the higher end of the ultra-high-energy cosmic ray spectrum, we’re seeing heavier atomic nuclei: not just hydrogen and helium, but heavy metals like iron. With ~56 protons and neutrons in each iron nucleus, the energy limit can exceed ~10²¹ electron-Volts, agreeing with observations at last.
When you bring all of this information together, it paints a startling picture of the Universe. Cosmic ray particles not only exist, but many of them come with energies that are millions of times greater than we can produce in the most powerful particle accelerators on Earth. Most of these particles are protons, but a few are composed of heavier atomic nuclei. At progressively higher energies, we see fewer and fewer particles, but at one particular critical energy — 5 × 10¹⁹ electron-Volts, corresponding to the energy where protons and Big Bang photons can produce pions — there’s a major dropoff, but higher-energy particles still exist.
After decades of mystery, we think we know why: the small fraction of heavier atomic nuclei can survive the journey through intergalactic space at these high energies, while protons cannot. With its energy spread out over ~50 or ~60 particles, these heavy, ultra-energetic composite particles can survive for many millions or even billions of years in space. While we still aren’t sure how they’re created, we can hang our hats on this accomplishment: we’ve at least solved the mystery of what these extreme cosmic particles are, and with it, their survival makes sense as well.
Starts With A Bang is written by Ethan Siegel, Ph.D., author of Beyond The Galaxy, and Treknology: The Science of Star Trek from Tricorders to Warp Drive.