How Close Are We To The Holy Grail Of Room-Temperature Superconductors?
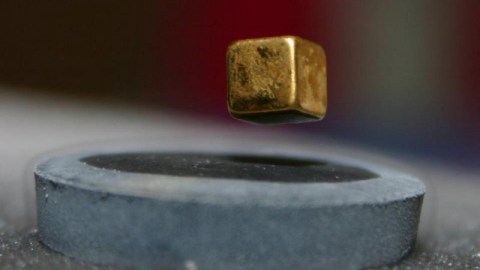
The dream of zero resistance is closer than you may think.
One of the biggest physical problems in modern society is resistance. Not political or social resistance, mind you, but electrical resistance: the fact that you cannot send an electrical current through a wire without some of that energy getting lost, being dissipated into heat. Electrical currents are just electric charges that move over time, and are harnessed by humans to move through current-carrying wires. Yet even the best, most effective conductors — copper, silver, gold, and aluminum — all have some resistance to current passing through them. No matter how wide, shielded, or unoxidized these conductors are, they’re never 100% efficient at transporting electrical energy.
Unless, that is, you can make your current-carrying wire go from a normal conductor to a superconductor. Unlike normal conductors, where the resistance gradually lowers when you cool them down, a superconductor has its resistance plummet to zero below a certain critical threshold. Without any resistance, superconductors can transmit electrical energy in a lossless fashion, leading to the holy grail of energy efficiency. Recent developments have brought about the highest-temperature superconductor ever discovered, but we probably won’t be transforming our electronics infrastructure anytime soon. Here’s the science of what’s going on at the frontiers.
Superconductivity has a long and fascinating history. We realized back in the 19th century that all materials — even the best conductors — still exhibit some sort of electrical resistance. You can lower the resistance by increasing the cross-section of your wire, by lowering the temperature of your material, or by decreasing the length of your wire. However, no matter how thick you make your wire, how cold you cool your system, or how short you make your electric circuit, you can never achieve infinite conductivity with a standard conductor for a surprising reason: electrical currents create magnetic fields, and any change in your resistivity will change the current, which in turn will change the magnetic field inside your conductor.
Yet perfect conductivity requires that the magnetic field inside your conductor not change. Classically, if you do anything to decrease the resistance of your conducting wire, the current will increase, and the magnetic field will change, meaning you can’t achieve perfect conductivity. But there’s an inherently quantum effect — the Meissner effect — that can arise for certain materials: where all magnetic fields inside a conductor are expelled. This makes the magnetic field inside your conductor zero for any current that flows through it. If you expel your magnetic fields, your conductor can begin behaving as a superconductor, with zero electrical resistance.
Superconductivity was discovered way back in 1911, when liquid helium first came into widespread use as a refrigerant. Scientist Heike Onnes was using liquid helium to cool down the element mercury into its solid phase, and was then studying the properties of its electrical resistance. Just as expected, for all conductors, the resistance gradually dropped as the temperature dropped, but only up until a point. Abruptly, at a temperature of 4.2 K, the resistance completely disappeared. Moreover, there was no magnetic field present inside the solid mercury once you crossed below that temperature threshold. Later only, several other materials were shown to exhibit this superconductivity phenomenon, all becoming superconductors at their own unique temperatures:
- lead at 7 K,
- niobium at 10 K,
- niobium nitride at 16 K,
and many other compounds subsequently. Theoretical advances accompanied them, helping physicists understand the quantum mechanisms that cause materials to become superconducting. After a series of experiments in the 1980s, however, something fascinating began to occur: materials composed of vastly different types of molecules not only exhibited superconductivity, but some did so at significantly higher temperatures than the earliest known superconductors.
It started with a simple class of materials: copper oxides. In the mid-1980s, experiments with copper oxides with the elements lanthanum and barium broke the longstanding temperature record by several degrees, being found to superconduct at temperatures greater than 30 K. That record was quickly broken by using strontium instead of barium, and then was broken once again — by a significant margin — by a new material: Yttrium-Barium-Copper-Oxide.
This wasn’t just a standard advance, but rather a huge leap: instead of superconducting at temperatures below ~40 K, which meant that either liquid hydrogen or liquid helium was required, Yttrium-Barium-Copper-Oxide became the first material discovered to superconduct at temperatures above 77 K (it superconducts at 92 K), meaning that you could use the much cheaper liquid nitrogen to cool your device down to superconducting temperatures.
This discovery led to an explosion of superconductivity research, where a variety of materials were introduced and explored, and not only extreme temperatures but also extreme pressures were applied to these systems. Despite the huge explosion in research surrounding superconductivity, however, the maximum superconductivity temperature stagnated, failing to crack the 200 K barrier (while room temperature is just a hair under 300 K) for decades.
Nevertheless, superconductivity has become incredibly important in enabling certain technological breakthroughs. It’s widely used in the creation of the strongest magnetic fields on Earth, which are all made through superconducting electromagnets. With applications ranging from particle accelerators (including the Large Hadron Collider at CERN) to diagnostic medical imaging (they’re an essential component of MRI machines), superconductivity isn’t just itself a fascinating scientific phenomenon, but one that enables some excellent science.
While most of us are probably more familiar with the fun and novel applications of superconductivity — such as using those strong magnetic fields to levitate frogs or taking advantage of superconductivity to make frictionless pucks levitating above and sliding across magnetic tracks — that’s not really the societal goal. The goal is to create an electrified infrastructure system for our planet, from power lines to electronics, where electrical resistance is a thing of the past. While some cryogenically cooled systems currently leverage this, a room-temperature superconductor could lead to an energy-efficiency revolution, as well as infrastructure revolutions in applications such as magnetically levitated trains and quantum computers.
In 2015, scientists took a relatively simple molecule — hydrogen sulfide (H2S), a molecule very analogous to water (H2O) — and applied an incredible pressure to it: 155 gigapascals, which is over 1500000 times the pressure of Earth’s atmosphere at sea level. (For comparison, this would be like applying more than 10,000 tonnes of force to every square inch of your body!) For the first time, the 200 K barrier was cracked, but only under these extremely pressurized conditions.
This line of research was so promising that many physicists who had become disillusioned with the prospect of achieving a practical solution to the superconductivity questioned took it up once again with renewed interest. In the October 14, 2020 issue of Nature, University of Rochester physicist Ranga Dias and his colleagues mixed hydrogen sulfide, hydrogen, and methane under extreme pressures: ~267 gigapascals, and were able to create a material — a “photochemically transformed carbonaceous sulfur hydride system” — that shattered the temperature record for superconductors.
For the first time, a maximum superconducting transition temperature of 288 K was observed: about 15 degrees Celsius or 59 degrees Fahrenheit. A simple refrigerator or heat pump would suddenly make superconductivity possible.
Last year’s discovery represented a tremendous symbolic breakthrough, as the increase in known superconducting temperatures followed a steady progression in recent years under extreme pressures. The 2015 work in pressurizing hydrogen and sulfur cracked the 200 K barrier, and 2018 research in a high-pressure compound involving lanthanum and hydrogen cracked the 250 K barrier. The discovery of a compound that can superconduct at liquid water temperatures (albeit at extremely high pressures) isn’t exactly a surprise, but it is a really big deal to break the room temperature barrier.
However, it seems that practical applications remain significantly far off. Achieving superconductivity at mundane temperatures but extreme pressures is not significantly more accessible than achieving it at mundane pressures but extreme temperatures; both are barriers to widespread adoption. In addition, the superconducting material only persists as long as the extreme pressures are maintained; once the pressure drops, so does the temperature at which superconductivity occurs. The next big step — one that remains to be taken — is to create a room temperature superconductor without these extreme pressures.
The concern is that there may be some sort of a Catch-22 situation at play here. The highest-temperature superconductors at standard pressures don’t appreciably change in behavior as you vary the pressure, while the ones that superconduct at even higher temperatures under high pressures no longer do so when you reduce the pressure. Solid materials that are good for making wires out of, like the various copper oxides discussed earlier, are very different than the pressurized compounds that are only created in trace quantities under these extreme laboratory conditions.
But — as first reported by Emily Conover at Science News — it’s possible that theoretical work, aided by computational calculations, could help point the way. Each possible combination of materials can give rise to a unique set of structures, and this theoretical and computational search can help identify which structures may be promising for obtaining the desired properties of high-temperature but also lower-pressure superconductors. The 2018 advance that crossed the ~250 K superconducting barrier for the first time, for example, was based on such calculations, which led to the lanthanum-hydrogen compounds that were then experimentally tested.
Already, such calculations have pointed towards a substantial advance by leveraging a new set of compounds: yttrium and hydrogen, which superconduct at near-room temperatures (-11 Celsius, or 12 Fahrenheit) but at substantially lower pressures than were previously required. While metallic hydrogen — which only exists at ultra-high pressures, such as those found at the bottom of Jupiter’s atmosphere — is expected to be an excellent high-temperature superconductors, the addition of extra elements could lower the pressure requirements while still maintaining the high-temperature superconductivity property.
Theoretically, all single-element combinations with hydrogen have now been explored for superconductivity properties, and the hunt is now on for two-element combinations, such as the carbon-sulfur-hydrogen compound previously discovered experimentally by Dias. Lanthanum and boron with hydrogen has shown promise experimentally, but the number of possible two-element combinations rises into the thousands. Only with computational methods can we receive guidance on what we ought to try next.
The biggest questions surrounding high-temperature superconductivity now all involve the pathway to getting to low pressures as well. The true “holy grail” moment will come when mundane conditions — in both temperature and pressure — can create a situation where superconductivity still persists, enabling a wide variety of electronic devices to leverage the power and promise of superconductors. Although individual technologies will advance, from computers to maglev devices to medical imaging and much more, perhaps the biggest benefits will come from the savings of vast amounts of energy in the electrical grid. High-temperature superconductivity, according to the US Department of Energy, could save the United States alone hundreds of billions of dollars in energy distribution costs annually.
In a world of finite energy resources, the elimination of any inefficiencies can benefit everyone: energy providers, distributors, and consumers at all levels. They can eliminate problems such as overheating, greatly reducing the risk of electrical fires. And they can also increase the lifespan of electronic devices while simultaneously reducing the need for heat dissipation. Once a novelty, superconductivity leapt into the scientific mainstream with the 20th century’s advances. Perhaps, if nature is kind, it will leap into the consumer mainstream with 21st century advances. Impressively, we’re already well on our way.
Starts With A Bang is written by Ethan Siegel, Ph.D., author of Beyond The Galaxy, and Treknology: The Science of Star Trek from Tricorders to Warp Drive.