Guest Post: How Astronomy Supports Evolution
How the Universe tells us its age, size, and properties, and leads us inescapably to the conclusion that it’s billions, not merely thousands, of years old.
Today, we’re lucky enough to have a guest post from Brian Koberlein: scientist, professor and science communicator extraordinaire. You can find Brian on his blog and on Google+.
A recent Pew survey has found that one third of Americans believe that humans and other living things have existed in their present form since the dawn of time. That’s one third of the adult population who reject evolution, which is the bedrock theory of biology. Indirectly, they also reject the foundations of geology, physics and astronomy. Much of the commentary about this survey has focused on the religious and political correlations, but let’s look at the science behind the ideas. If evolution is correct (and it is) then it must have occurred over billions of years, not a mere 10,000 or so. So how do we know — really, really know — that the Universe is billions of years old? It all comes down to a bit of astronomy.
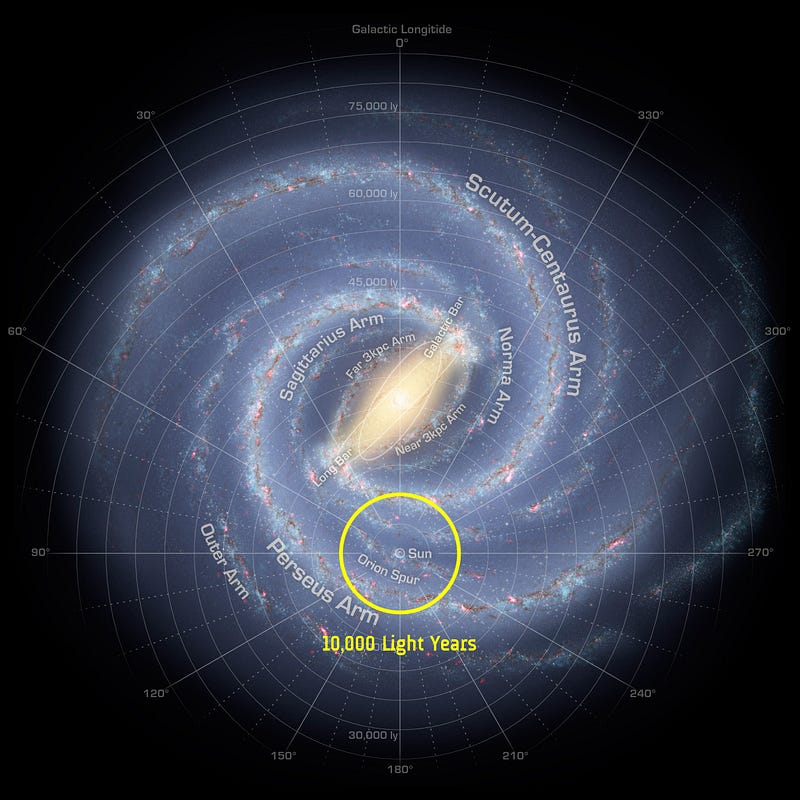
One way we determine the age of the Universe is through cosmic distances. Since light travels at a finite speed, the light from distant objects takes time to reach us. The more distant the objects we can see, the older the Universe must be. So how far does 10,000 years get you? Not very far, as you can see in the figure above. For anything outside the yellow circle, the light has taken longer than 10,000 years to reach us. If the Universe was only 10,000 years old, we wouldn’t yet see anything beyond that circle. The faint glow of the Milky Way in a dark sky? Most of it would be missing. The Large Magellanic Cloud? Totally gone. The Andromeda galaxy? Not a chance. The night sky of a young Universe would be darker, and not nearly as interesting.
So how do we know our distances are correct? There are actually several methods to determine cosmic distances, and these are combined to create what is known as the cosmic distance ladder. The most direct method uses the property of parallax. Parallax occurs when you look at an object from two slightly different positions. You probably use it every day, because it is what gives humans depth perception. When you look at an object, each of your eyes has a slightly different point of view. Your brain uses this information to determine which objects are close and which are farther away. This is also why you have to wear special glasses when you go to see a 3D movie. The glasses ensure that your eyes each get a slightly different perspective, which gives the movie the illusion of depth. If you take off the glasses during the movie, it will look slightly blurry. Without the glasses, your eyes see both points of view blurred together.
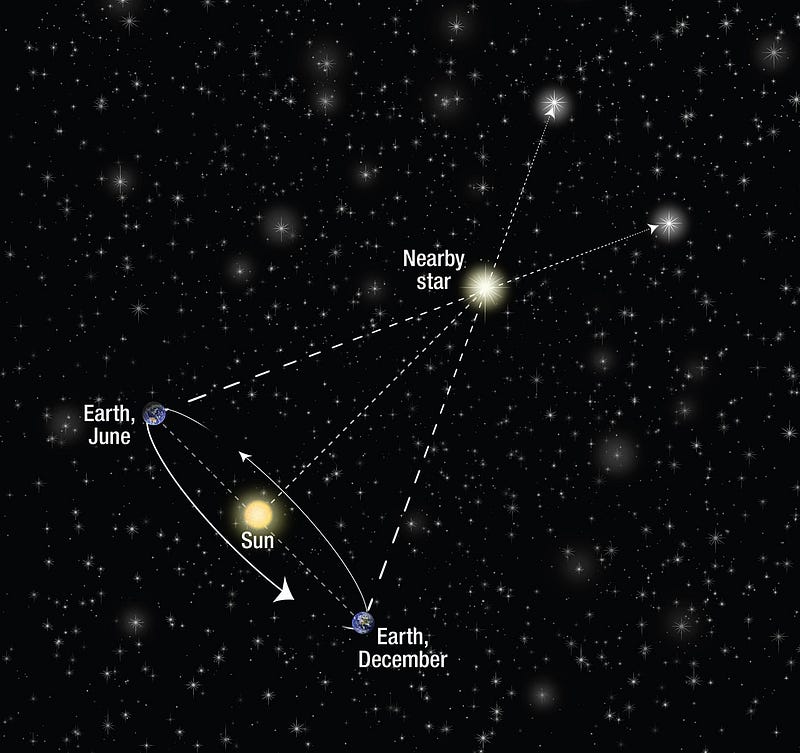
You can see the effect of parallax with a simple experiment. Hold up your thumb at arm’s length, and look at it with only one eye. Without moving your thumb, switch eyes, and you will see that your thumb appears to move relative to more distant objects. This shift is known as a parallax shift. If you bring your thumb closer and do the experiment again, you’ll see that the parallax shift is larger. If it is farther away, the parallax shift is smaller.
With a little bit of trigonometry, you can calculate the distance to an object by measuring its parallax. This is how astronomers can measure the distances to nearby stars, using the motion of the Earth to their advantage. The radius of the Earth’s orbit about the Sun is 150 million kilometers. By observing the position of a star on a particular night, and then on a night months later, astronomers can measure the parallax shift of the star from two points of view. The bigger the parallax shift, the closer the star. The recently launched Gaia spacecraft can measure parallax with a precision of a few microarcseconds, which gives us the ability to measure stellar distances up to 30,000 light years away with an accuracy of 10%.
Beyond that distance parallax is too small to be of use, so we can use another method looking at a type of star known as a cepheid variable. Cepheid variables are stars that vary in brightness over a period of days. The first such star to be observed was Delta Cephei in 1784 (the fourth brightest star in the constellation of Cepheus), hence the name. For nearby Cepheids, we can determine their distance via parallax. We can also determine their apparent magnitude (how bright they appear), and given their distance we can determine their absolute magnitude (how bright they actually are) using the fact that the brightness of an object decreases with distance following what is known as an inverse square law.
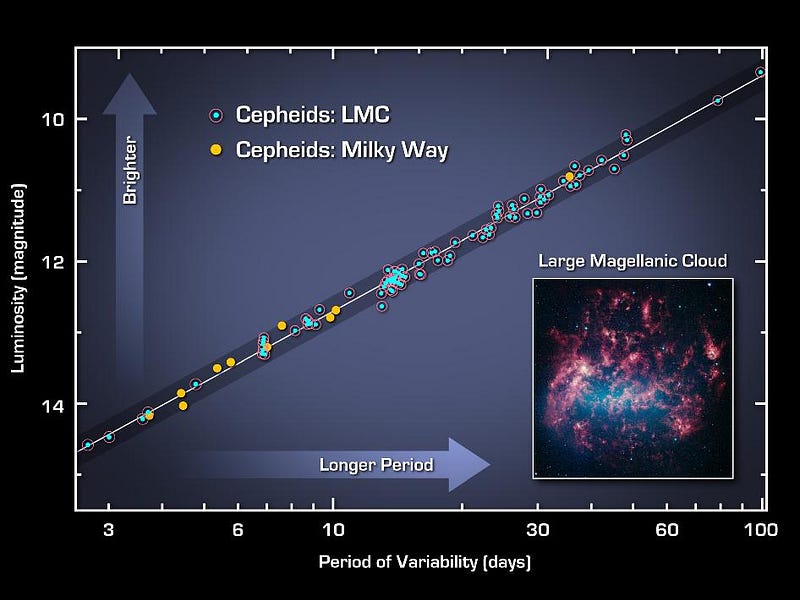
In in the early 1900s astronomer Henrietta Leavitt analyzed more than 1700 variable stars to discover the luminosity-period relation for Cepheid variables. By looking at Cepheids in a particular Magellanic cloud she was able to demonstrate a linear relationship between absolute brightness (luminosity) and period, such as seen in the figure above. This meant Cepheids could be used as “standard candles”. By observing their variable period, we can determine their absolute brightness. Comparing this to their apparent brightness, we can determine their distance. From the Hubble telescope we have observations of Cepheid variables in lots of nearby galaxies, for which we can measure galactic distances out to about 100 million light years.
Beyond this distance, Cepheid variables are too faint to use accurately, so we need another method. This is often done with another class of standard candle known as a Type Ia Supernova. This type of supernova can often occur when two white dwarfs are in close orbit with each other. A white dwarf is formed when a Sun-sized star begins to run out of hydrogen to fuse in its core. The star fuses helium for a while, causing it to swell into a red giant. Depending on its mass, a star will fuse some higher elements in its core, and the resulting heat and light drives off much of the outer material of the star, but there comes a point where the star simply can’t keep fusing higher elements. After this, what remains of the star compresses down to a white dwarf. In a white dwarf it isn’t the heat and pressure of fusion that balances against the weight of gravity, but the pressure of the electrons pushing against each other. Type Ia Supernova are typically caused by a collision or merger of two white dwarfs. If the two stars are in a close binary orbit, particularly with a third star orbiting at as part of a trinary system, the orbits of the white dwarfs can degrade to the point where they collide, resulting in a supernova explosion.
What makes these type of supernovae particularly interesting is that they always have about the same brightness. We’ve observed Type Ia Supernovae in galaxies whose distance was already known from the Cepheid variables. We can observe how bright the supernovae appear, and knowing their distance we can determine how bright they actually are. What we find is that Type Ia Supernovae always have the same luminosity.
This property means we can use them as a standard candle as well. If we observe a Type Ia Supernova in a distant galaxy, we can observe how bright it appears. Since we know how bright it actually is, we can calculate the distance to the galaxy, since the more distant a light source is, the dimmer it appears. We can therefore use this type of supernova to measure the distance to its galaxy. This allows us to measure cosmic distances of billions of light years.
Now, as a skeptic you might point out that all I’ve done is shown that the Universe is large, not that it is old. Sure, the light of distant galaxies might take billions of years to reach us now, but what if the speed of light were much faster in the past? How do we know that the speed of light hasn’t changed over time?
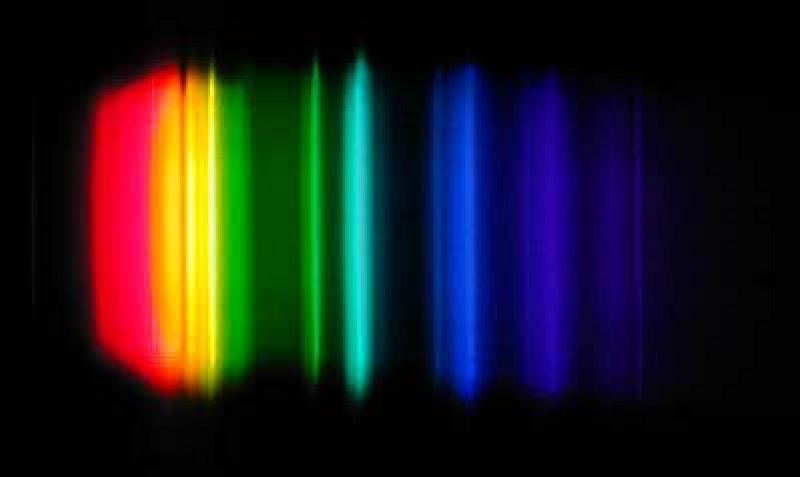
One of the things we can do is look at the emission and absorption spectra of atoms and molecules in distant stars, nebulae and galaxies. The patterns of these spectra allow us to identify these atoms and molecules, like a kind of fingerprint. But they also allow us to test whether physical constants have changed over time. Not just the speed of light, but the charge of the electron, Planck’s constant and others. If any of these constants had changed over time, the lines in a spectrum would shift relative to each other. The pattern would spread apart in some areas and scrunch together in others. When we look at distant objects, we find no such shift in any of them. Given the limits of our equipment, this means the speed of light can have changed no more than one part in a billion over the past 7 billion years. As far as we can observe, the speed of light has always been the same.
So this gives us confidence in a wonderful aspect of observational astronomy. When you look at more and more distant objects, you are also looking further back into time. But we can take that idea one step further, because not only do we know the Universe is old, we know just how old it is using the Doppler effect. The observed color of light can be affected by the relative motion of its source. If a light source is moving toward us, the light we see is more bluish than we would expect (blueshifted). If a light source is moving away from us, the light is more reddish (redshifted). The faster the source is moving, the greater the shift.
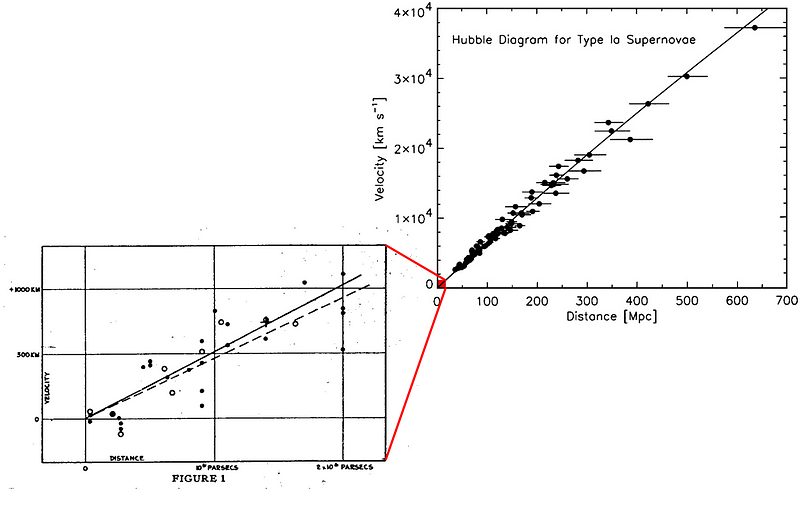
We’ve measured this color shift for lots of stars, galaxies and clusters, and when we plot a graph of the distance of galaxies versus their redshift we find an interesting relation, seen above. The greater a galaxy’s distance, the greater its redshift. This means galaxies are not simply moving at random, as you would expect in a stable, uniform Universe. Instead, the more distant the galaxy the faster it is moving away from us. This relation between distance and speed is the same in all directions, which means the Universe seems to be expanding in all directions. Of course if the Universe is expanding, then it must have been smaller in the past. In other words, the Universe has a finite age, and it began very small, very dense (and therefore very hot). We call that starting point the Big Bang. If you do the math, you get an age of about 13.8 billion years.
Of course the story I’ve told here is just one path to the age of the Universe. We have lots of other observational evidence such as the cosmic microwave background, stellar evolution, baryon acoustic oscillations, and the hydrogen/helium ratio, to say nothing of planetary science, geology, and biology. This confluence of evidence points to a Universe that is not thousands, but billions of years old.
There was a time when the idea of a small, young Universe seemed reasonable. We now know that it is far older and far more wondrous than we ever expected.
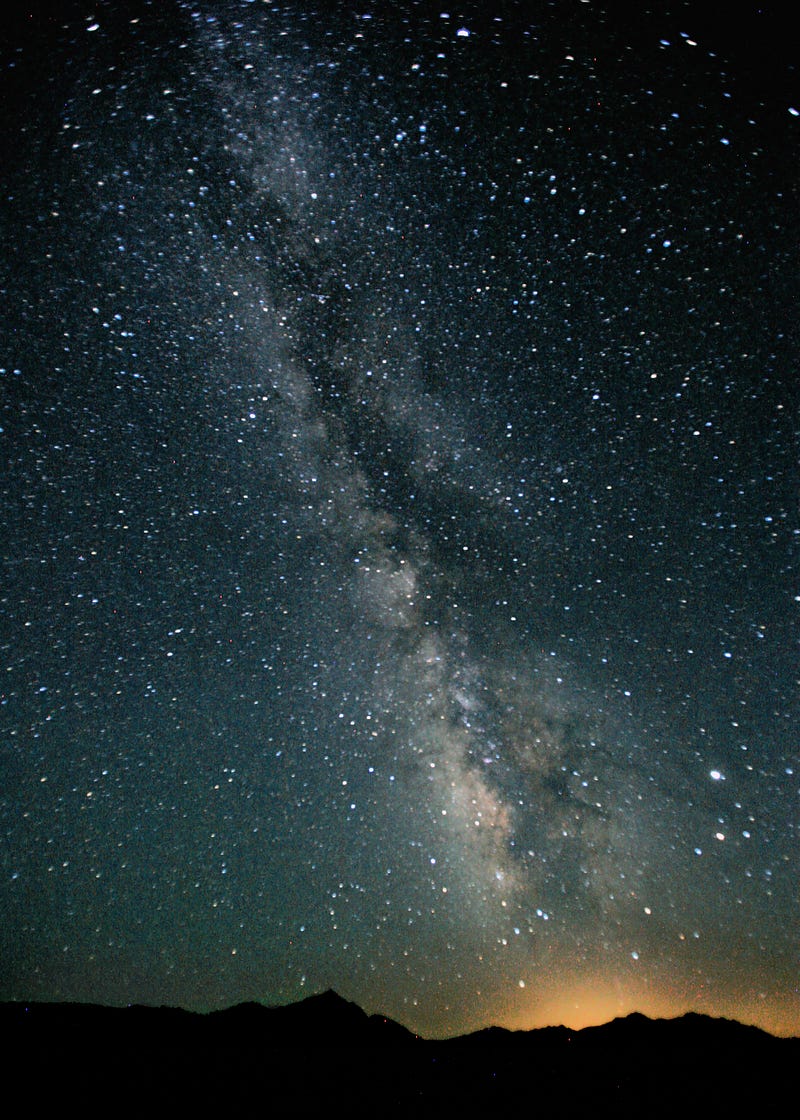