Dark Matter Search Discovers A Spectacular Bonus: The Longest-Lived Unstable Element Ever
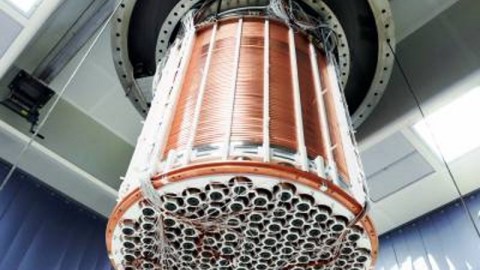
Xenon-124 isn’t stable, and the direct detection of its decay could lead us to an even greater prize.
Our Universe is old: 13.8 billion years old, to be precise. Many of the chemical elements that appear stable on short timescales will turn out to be fundamentally unstable, decaying away into other elements if we wait long enough. While many of these decays are easily observable, some elements and isotopes are so long-lived that their half-lives are greater than the age of the Universe.
In a spectacular discovery, the XENON collaboration has just publicly announced the discovery that xenon-124, an isotope of the element Xenon, is fundamentally unstable. Its half-life is a whopping 1.8 × 10²² years: more than one trillion times the present age of the Universe. It’s the longest half-life humanity has ever measured directly, and its implications for the nature of reality couldn’t be more profound.
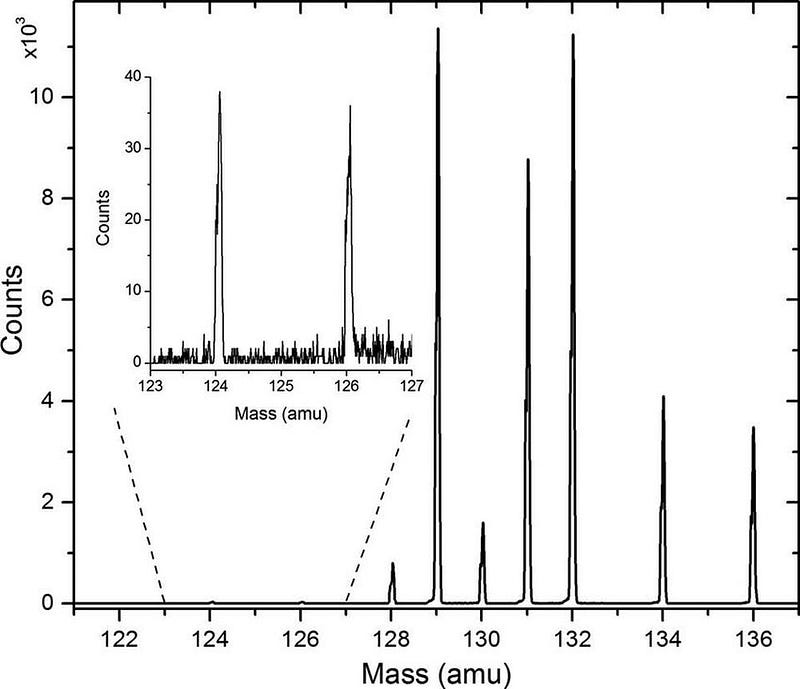
Every imaginable combination of protons and neutrons represents a possible isotope of an element on the periodic table. Some of these combinations are absolutely stable, such as carbon-12, which has six protons and six neutrons. Even if you waited an arbitrarily long time, the evidence thus far indicates that the carbon-12 nucleus will never decay.
But different combinations aren’t stable, and will spontaneously either emit or capture one or more particles, transforming into a different element or isotope in the process. Carbon-14, for example, contains six protons and eight neutrons. If we observe carbon-14 for long enough, we’ll find that it’s unstable: it will radioactively decay into nitrogen-14, emitting an electron and an antineutrino in the process.
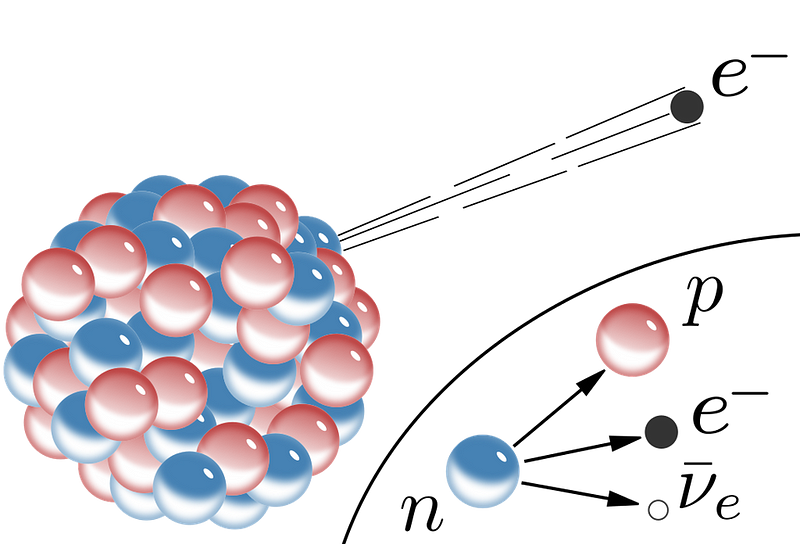
For those of us who learned about radioactivity prior to 2003, we were taught that each element containing more protons than bismuth (83) is fundamentally unstable. For elements like radium, thorium, radon, uranium and plutonium, every one of their isotopes undergoes radioactive decay.
In 2003, however, the world learned the truth about bismuth: it, too, is fundamentally unstable. There’s one isotope of bismuth, containing 83 protons and 127 neutrons, that was previously thought to be stable. But on timescales of 1.9 × 10¹⁹ years, it, too, will radioactively decay, emitting a helium nucleus and transmuting into thallium (the element before lead). If your periodic table is newer than that discovery, it indicates that lead — with 82 protons — is the heaviest stable element.
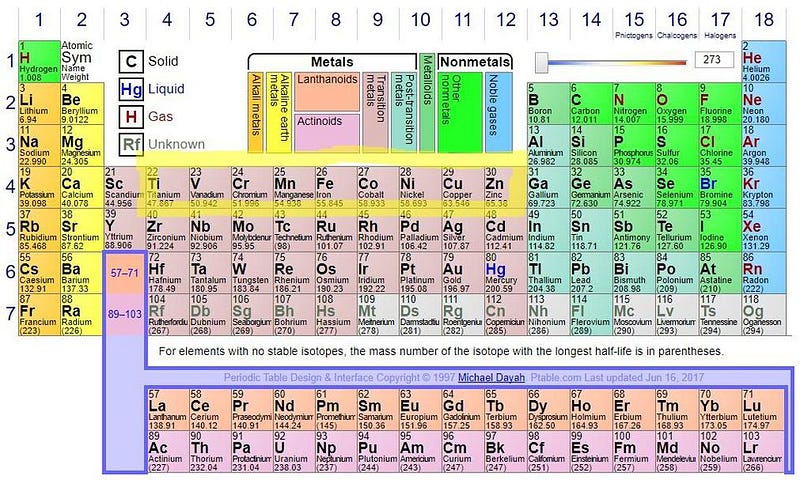
It sounds like a bizarre proposition: to measure a process that takes longer to occur than the age of the Universe. A single atom of bismuth will last, on average, more than one billion times longer than the Universe has been around for.
But we don’t measure radioactivity by watching a single atom; we take enormous collections of atoms and search for any telltale signature that even one of them decays. If we had a mole (6.022 × 10²³) of bismuth atoms, even with their massively long half-lives (the amount of time it takes half of the atoms to decay), we’d see tens of thousands of them decay away with every year that goes by.
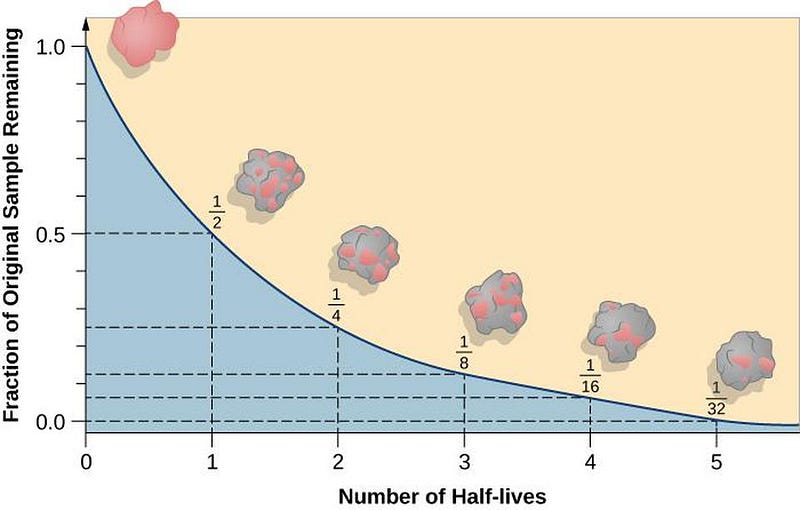
There are two extremely common ways for radioactive decay to occur:
- alpha decay, where an atomic nucleus emits an alpha particle (a helium nucleus), containing two protons and two neutrons, producing a new nucleus that’s two elements earlier on the periodic table,
- or beta decay, where an atomic nucleus emits an electron and an antineutrino, transforming one of its neutrons into a proton in the process, producing a new nucleus that’s one element higher on the periodic table.
Carbon-14 decays via beta decay; uranium-238 decays via alpha decay. So long as the combined masses of the decay products are lighter than the initial atomic nucleus, radioactive decay is possible.
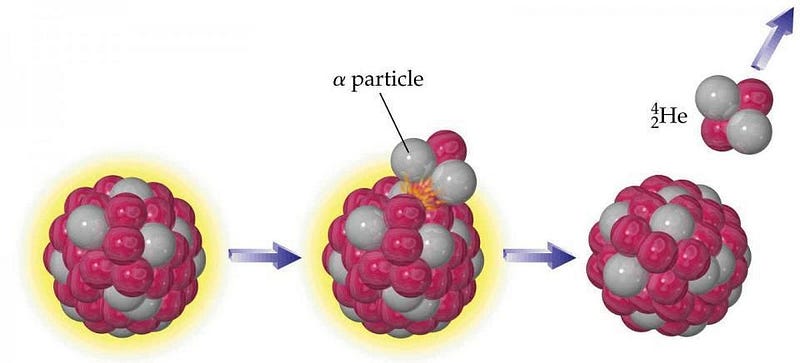
But there are even rarer decays that can occur, and they can be seen when the more common decay pathways are either suppressed or forbidden. Some nuclei undergo inverse beta decay: transforming a proton into a neutron by emitting a positron (the antimatter counterpart of the electron) and a neutrino, dropping down one element on the periodic table. Other nuclei drop down one element by capturing one of the innermost electrons orbiting it, turning a proton into a neutron and causing the emission of a neutrino.
Because there are subtle differences between oddly-charged and evenly-charged nuclei, sometimes double beta decay can occur where normal beta decay cannot, resulting in the emission of two electrons and two antineutrinos. And, in the rarest type of known decay of all, we can have double electron capture: where two electrons are simultaneously captured by the atomic nucleus.
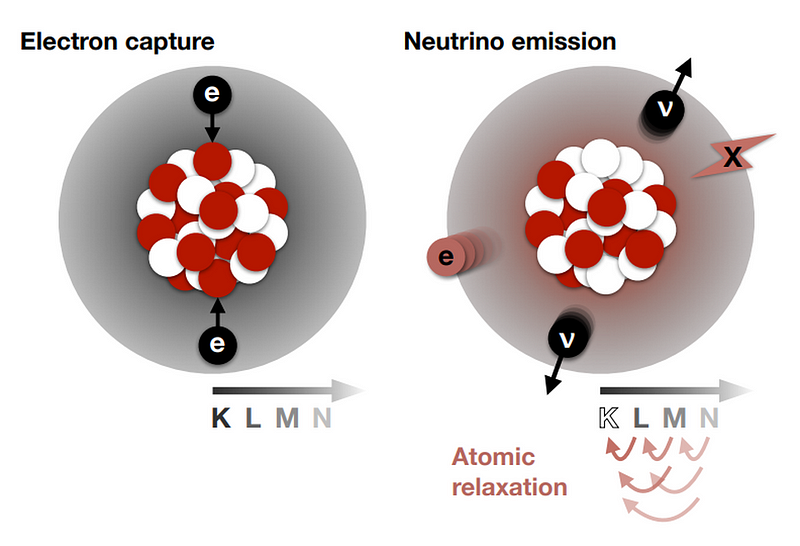
Up until now only two known isotopes in nature — krypton-78 and barium-130 — have been shown to transmute via double electron capture. In both cases, neither of the two emitted neutrinos can be detected, nor can the minute recoil of the nucleus. Instead, it’s the effects of the electrons that cascade down in energy that we can detect. As the electrons transition to lower energy levels to fill those gaps resulting from the earlier electron capture, they emit X-rays and also cause surrounding electrons to become free and unbound.
That’s where having an ultra-sensitive detector comes in. You want to be able to both detect the X-rays at the pinpoint location of their creation, and also to observe how the newly-freed electrons drift when you apply an external field. Through the detection of both secondary signatures, which is only possible in an extraordinarily pristine environment, we can reconstruct what happened inside the detector, as well as where and when.
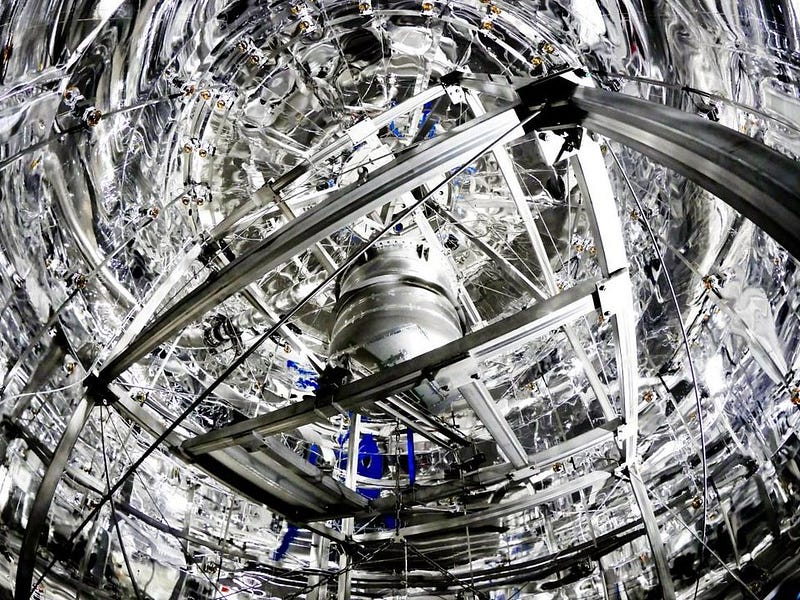
The XENON collaboration possesses exactly the type of environment that should be sensitive to rare processes like these. Designed to uncover the signature of any dark matter particle that might pass through the detector and collide with a xenon nucleus, the XENON collaboration has placed some of the strongest limits on dark matter’s interaction cross-sections with normal matter in history. In order to look for these detections, they have to understand and eliminate their backgrounds in a superior, never-before-achieved fashion.
According to postdoc Laura Manenti, a member of XENON’s public relations team:
it shows how low in background our detector is, which means we have the capability to build technology able to find the elusive dark matter.
Well, dark matter hasn’t yet been found by XENON, but something remarkable has.
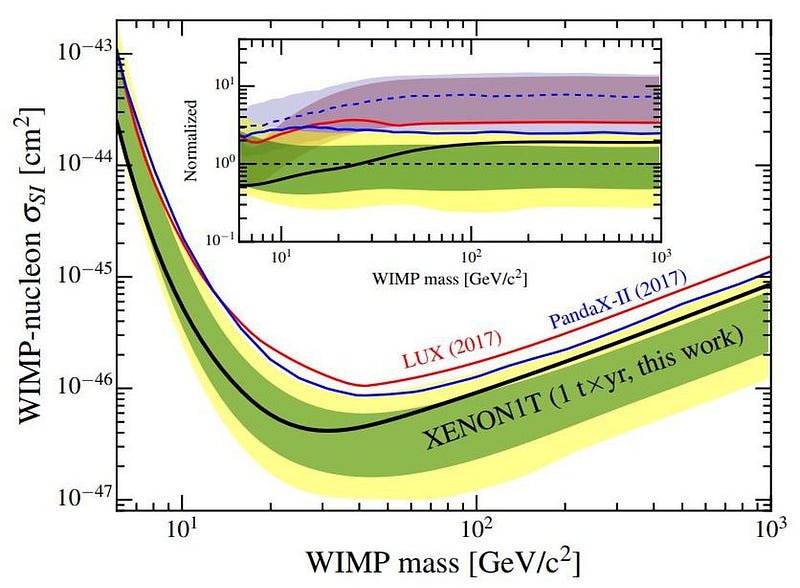
You see, the way the XENON detector works is by arranging a large amount of xenon — the inert, non-interacting gas whose nucleus has 54 protons — inside one of the world’s most well-shielded, sophisticated detectors. Although it’s called the XENON1T detector, there are actually 3,200 kg of xenon inside. Many of xenon’s most sensitive interactions can be revealed, including the possibility of finding processes and decays that have never been seen before. The ultimate goal of this quest, though, is to reveal the presence (or constrain the properties) of dark matter.
Xenon, naturally, comes in not one but nine different isotopes, with the lightest being xenon-124 (with 70 neutrons) and the heaviest being the long-lived but unstable xenon-136, which undergoes double beta decay after around 2 × 10²¹ years. Of the other eight isotopes, they have always been observed to be stable, but three of them are theoretically expected to undergo double electron capture. It’s just never been observed.
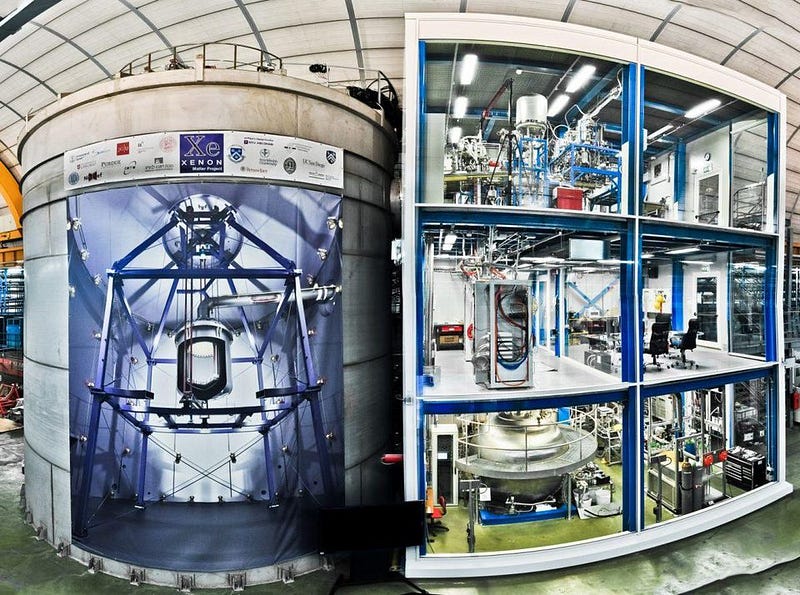
Until, that is, the latest run of the experiment! From 2016 until 2018, the XENON collaboration monitored and collected observations involving everything that occurred inside the detector. One of the surprising signals they found were of x-rays emitted from a particular point, followed by free electrons drifting up and triggering the detector with a slight delay. There were a total of 126 events that correspond to this process, with the energy matching the theoretical predictions of the double electron capture of one of xenon’s isotopes: xenon-124.
With a paper accepted by the prestigious journal Nature (to be published on April 25), the XENON collaboration has now broken the record for measuring the longest-lived decay in history. With a half-life of 1.8 × 10²² years, the double electron capture process of xenon-124 has both revealed the incredible sensitivity of the detector, and demonstrated the importance of looking past the known frontiers of science.
It’s also a testament to the contributions of the collaboration’s members who add a wide variety of skills and specialties. “Observing such a rare process would not have been possible without the joint work of analysers as well as of the people who have built and operated the detector,” according to scientist Christian Wittweg, a coauthor on the discovery paper. “It is a big collaborative effort!”
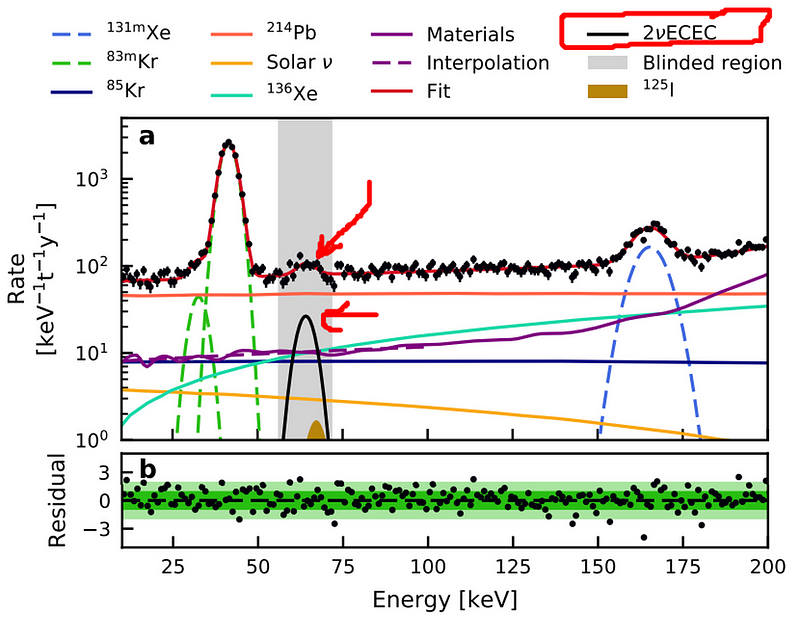
Whenever you build an experiment that can take you beyond your previous sensitivity limits, you open yourself up to the possibility of discovery. In robustly detecting this extraordinarily rare decay with a longer lifetime than any other we’ve ever seen, the XENON collaboration has demonstrated how capable their apparatus is. Although it was designed to search for dark matter, it’s also sensitive to a number of other possibilities which might herald rare or even entirely new physics.
While the direct detection of the longest-lived unstable decay is an incredible feat, its implications go far beyond a simple discovery. It’s a demonstration of XENON’s sensitivity, and its ability to tease out even a tiny signal against a well-understood, low-magnitude background. It gives us every reason to be hopeful that, if nature is kind, XENON may reveal some of its even more profound secrets.
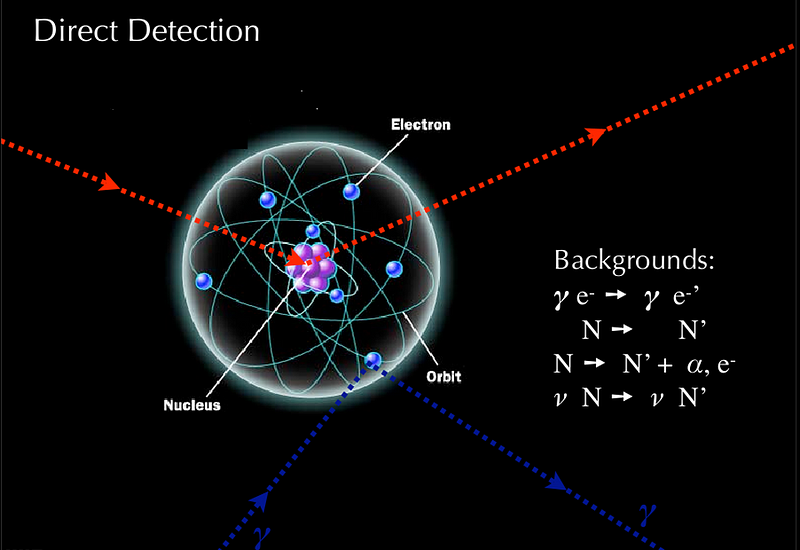
With the rarest double electron capture decay under their belt, the XENON collaboration is now looking ahead to other possibilities, such as neutrinoless double electron capture or neutrinoless double beta decay, which could both occur if the neutrino has certain special properties that make it its own antiparticle: that of a Majorana fermion.
The XENON detector is currently being upgraded to even greater precision, where perhaps new decays and properties of nature will be revealed. Will other isotopes of xenon be discovered to exhibit double electron capture? Will neutrinoless double electron capture or neutrinoless double beta decay show up? Will the direct signatures of dark matter be revealed at long last?
With this latest discovery, there’s every reason to believe that whatever the natural truths of our reality are, the XENON collaboration will help reveal them.
The author credits Nature and scientist Laura Manenti as essential sources of information used in putting this story together.
Ethan Siegel is the author of Beyond the Galaxy and Treknology. You can pre-order his third book, currently in development: the Encyclopaedia Cosmologica.