Why “chaos and complex systems” absolutely deserves 2021’s Nobel Prize in physics
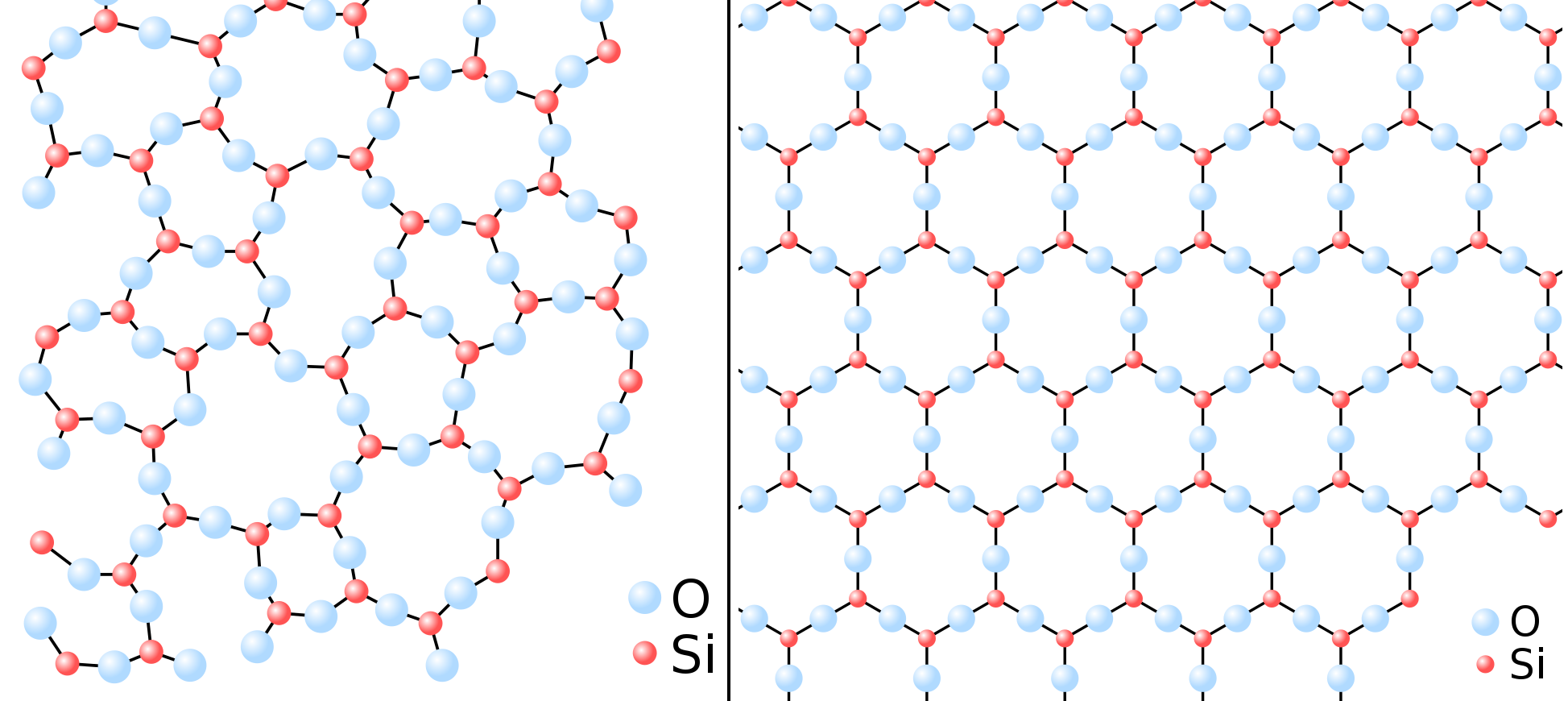
- In science, we attempt to model systems as simply as possible, without losing the relevant effects.
- But for complex, interacting, many-particle systems, it takes a herculean effort to extract the needed behavior to make meaningful predictions.
- 2021’s Nobel laureates in physics — Klaus Hasselmann, Syukuro Manabe, and Giorgio Parisi — all revolutionized their fields in exactly this fashion.
One of the oldest jokes in physics is that you should begin by imagining a spherical cow. No, physicists don’t think that cows are spherical; we know this is a ridiculous approximation. However, there are cases where it’s a useful approximation, as it’s much easier to predict the behavior of a spherical mass than a cow-shaped one. In fact, as long as certain properties don’t really matter for the sake of the problem you’re trying to solve, this simplistic view of the universe can help us arrive at accurate-enough answers quickly and easily. But when you go beyond single, individual particles (or cows) to chaotic, interacting, and complex systems, the story changes significantly.
For hundreds of years, before even the time of Newton, we approached problems by modeling a simple version of it that we could solve and then modeling additional complexity atop it. Unfortunately, this type of oversimplification causes us to miss out on the contributions of multiple important effects:
- chaotic ones that arise from many-body interactions extending all the way to the system’s boundaries
- feedback effects that arise from the evolution of the system further affecting the system itself
- inherently quantum ones that can propagate throughout the system, rather than remaining confined to a single location
On October 5, 2021, the Nobel Prize in physics was awarded to Syukuro Manabe, Klaus Hasselmann, and Giorgio Parisi for their work on complex systems. While it might seem like the first half of the prize, going to two climate scientists, and the second half, going to a condensed matter theorist, are completely unrelated, the umbrella of “complex systems” is more than big enough to hold them all. Here’s the science of why.
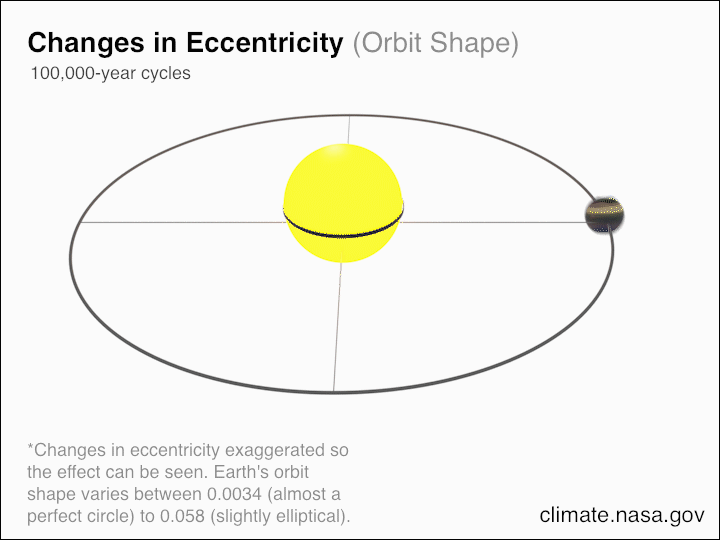
Imagine, if you will, that you have a very simple system: a particle moving in a circle. There are a variety of physical reasons why a particle could be compelled to move along a continuous circular path, including:
- the particle is part of a rotating circular body, like a vinyl record,
- the particle is being attracted towards the center while moving, like a planet orbiting the sun,
- or the particle is confined to a circular track, and is prohibited from taking any other path.
Regardless of the particulars of your setup, it would be completely reasonable to assume that if you had many versions (or copies) of this system all coupled together, you’d simply see the behavior of that one simple system repeated many times. But this isn’t necessarily the case, because each simple system can interact with every other simple system and/or with the environment, leading to a vast array of possible outcomes. In fact, there are three main ways that a many-body system can exhibit complex behavior in a way that a simple, isolated system cannot. In order to understand what 2021’s Nobel Prize in physics is all about, here are the three things we need to keep in mind.
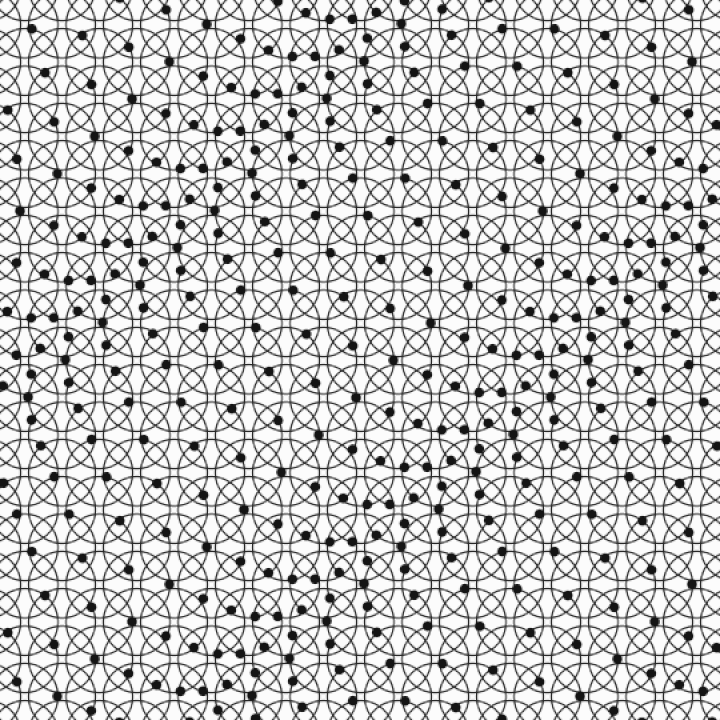
Credit: Dave Whyte/Bees & Bombs
1.) Complex systems can exhibit aggregate behaviors that only emerge from the interaction of many smaller, simpler systems. It’s a remarkable feat that we can take the same simple system we were just considering — a particle moving along a circular path — and, by combining enough of them, can observe a complex, aggregate behavior that no individual “part” would reveal. Even if the circular path that each particle takes is static and unmoving, as above, the collective behaviors of each component, when taken together, can sum up to something spectacular.
In realistic physical systems, there are certain properties that remain fixed even while others evolve. The fact that certain properties remain unchanged is no indication that the whole system will remain constant, however; properties that change in one location can lead to dramatic changes that can occur elsewhere or overall. The key is to make as many simplifying approximations as possible without oversimplifying your model and running the risk of losing or altering the relevant behavior. Although this is no easy task, it’s a necessary one if we want to understand the behavior of complex systems.
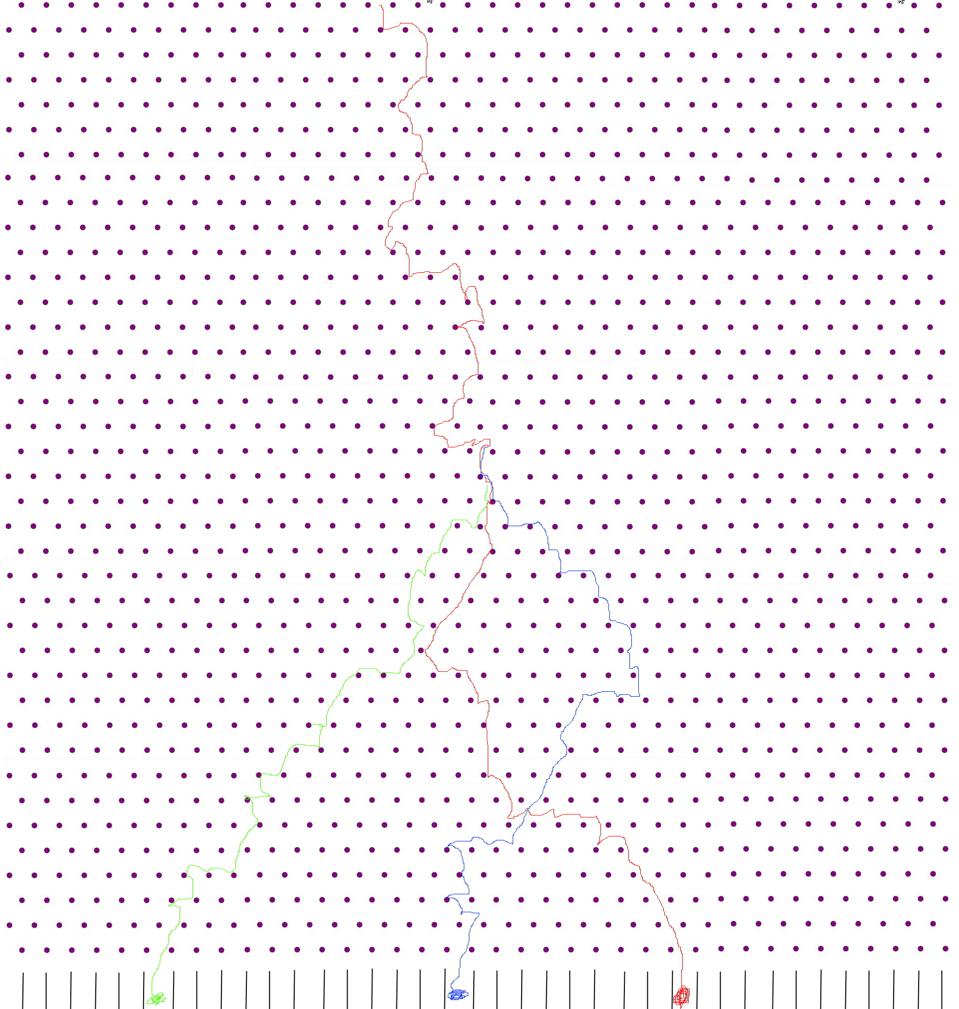
(Credit: E. Siegel)
2.) Small changes to the conditions of a system, either initially or gradually over time, can lead to wildly different outcomes in the end. This is no surprise to anyone who’s swung a double pendulum, tried to roll a ball down a mogul-filled slope, or dropped a Plinko chip down a Plinko board. Tiny, minuscule, or even microscopic differences in the speed or position of how you start your system off can lead to dramatically disparate outcomes. There will be a certain point up to which you can confidently make predictions about your system, and then a point beyond that where you’ve gone beyond the limits of your predictive power.
Something as small as reversing the spin of a single quantum particle — or, to take a more poetic viewpoint, the flapping of a distant butterfly’s wings — can be the difference between whether an atomic bond is broken, whose signals can then propagate to other adjacent atoms. Further downstream, this could be the difference between winning $10,000 or $0, whether a dam holds together or crumbles away, or whether two nations wind up going to war or remain at peace.
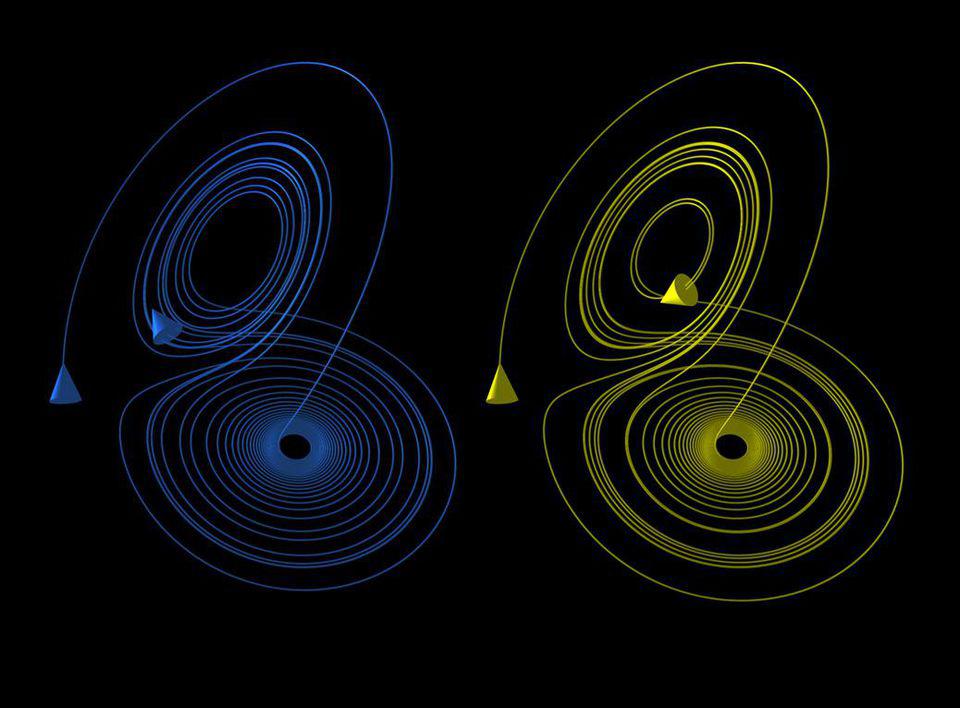
3.) Even though chaotic systems aren’t perfectly predictable, meaningful aggregate behavior can still be understood. This is maybe the most remarkable feature of chaotic, complex systems: Despite all of the uncertainties that are present and all of the interactions that occur, there is still a likely, predictable set of probabilistic outcomes that can be quantified. There are also some general behaviors that can sometimes be extracted, despite the intrinsic variability and the complexity of the system.
Keep these three things in mind:
- a complex system is many simpler components acting together,
- it is sensitive to initial conditions, evolution, and the system’s boundaries,
- despite the chaos, we can still make important, general predictions,
Now, we’re ready to dive into the science that underpins the 2021 Nobel Prize in physics.
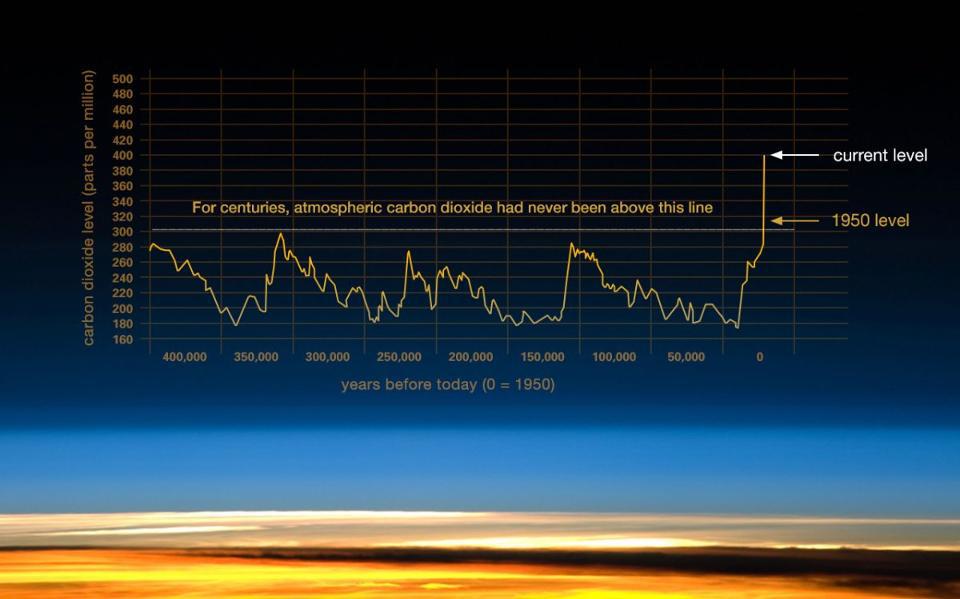
Earth’s climate is one of the most complex systems we routinely deal with. The incoming solar radiation strikes the atmosphere, where some light is reflected, some is transmitted, and some is absorbed, and then both energy and particles are transported, where heat is reradiated back into space. There’s an interplay between the solid earth, the oceans, and the atmosphere, as well as our incoming and outgoing energy budgets and the biological systems present on our world. You might suspect that this complexity would make any sort of end-to-end, cause-and-effect type of prediction extraordinarily difficult to extract. But Syukuro Manabe was perhaps the first to successfully do it for one of the most pressing problems facing humanity today: global warming.
In 1967, Manabe coauthored a paper with Richard Wetherald that connected the incoming solar and outgoing thermal radiation not only to the atmosphere and Earth’s surface, but also to:
- the oceans
- water vapor
- cloud cover
- the concentrations of various gases
Manabe and Wetherald’s paper not only modeled these components, but also their feedbacks and interrelationships, showing how they contribute to Earth’s overall average temperature. For example, as the atmospheric contents change, so do the absolute and relative humidity, which alter the total global cloud cover, affecting the water vapor content and the cycling and convection of the atmosphere.
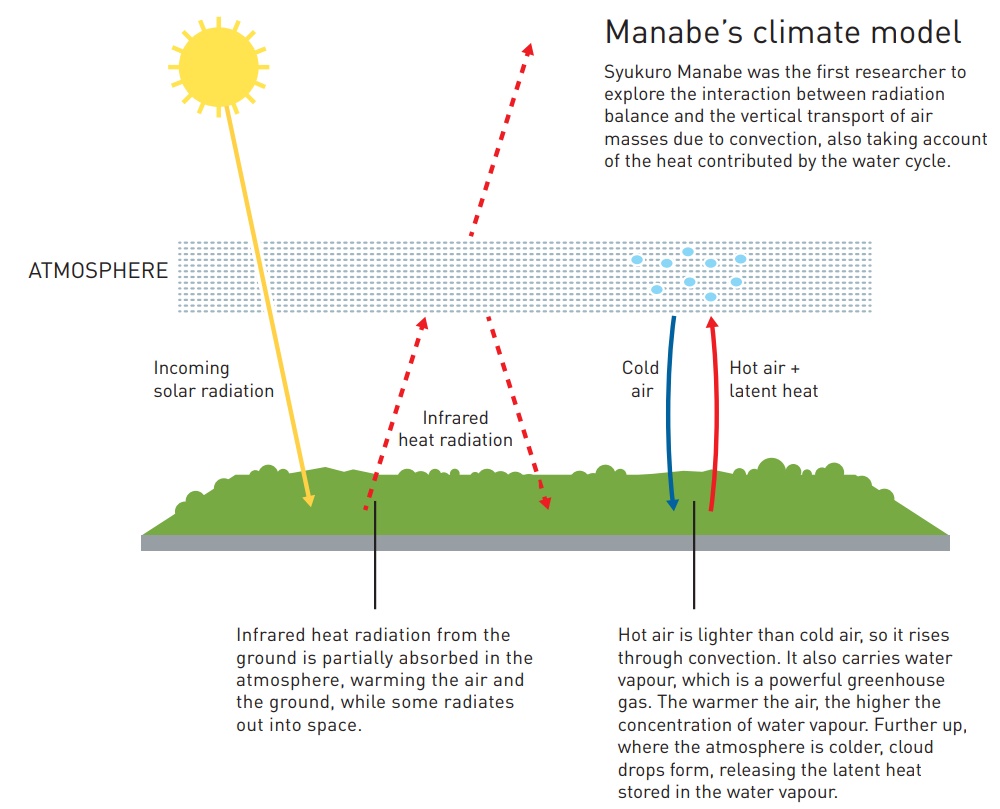
The enormous advance of the Manabe and Wetherald paper was to show that if you begin with an initially stable state — such as what Earth experienced for the thousands of years prior to the industrial revolution — you can tinker with a single component, like the CO2 concentration, and model how the remainder of the system evolves. (Wetherald died in 2011, so he was ineligible for the Nobel Prize.) Manabe’s first climate model successfully predicted the magnitude and time rate-of-change of Earth’s global average temperature as correlated with CO2 levels: a prediction that’s been borne out over more than a half-century. His work became the foundation for the development of today’s current climate models.
In 2015, the lead authors and review editors on that year’s IPCC report were asked to nominate their choices for most influential climate change papers of all time. The Manabe and Wetherald paper received eight nominations; no other paper received more than three. In the late 1970s, Klaus Hasselmann extended Manabe’s work by linking the changing climate to the chaotic, complex system of weather. Prior to Hasselmann’s work, many pointed to chaotic weather patterns as evidence that climate model predictions were fundamentally unreliable. Hasselmann’s work answered that objection, leading to model improvements, lowered uncertainties, and greater predictive power.
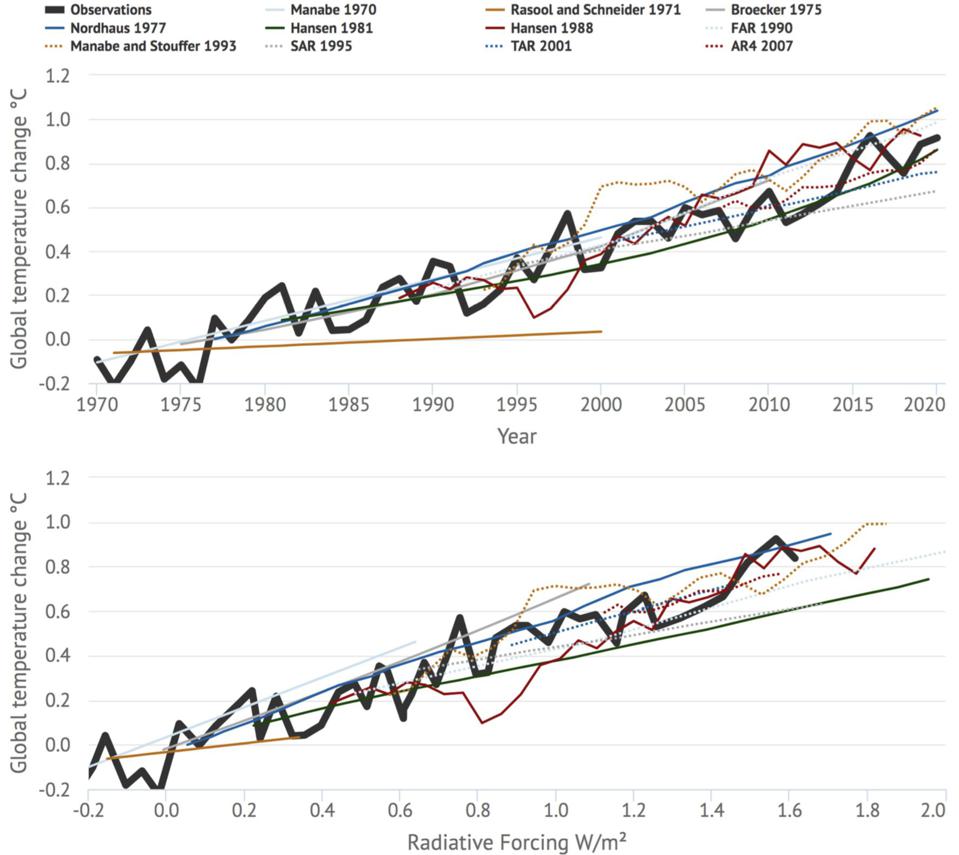
But perhaps the greatest advance that Hasselmann’s work enabled came from his methods for identifying the “fingerprints” that natural phenomena and human activity leave in the climate records. It was his methods that were leveraged to demonstrate that the cause of the recently increased temperatures in Earth’s atmosphere are due to the human-caused emission of carbon dioxide gas. In many ways, Manabe and Hasselmann are the two most important living scientists whose work paved the way to our modern understanding of how human activity has caused the ongoing and related problems of global warming and global climate change.
In a very different application of physics to complex systems, the other half of the 2021 physics Nobel Prize went to Giorgio Parisi for his work on complex and disordered systems. Although Parisi has made many vital contributions to a variety of areas in physics, the hidden patterns he discovered in disordered, complex materials are arguably the most important. It’s easy to imagine extracting the overall behavior of a regular, ordered system made up of individual components, like:
- stresses within a crystal
- compression waves traveling through a lattice
- the alignment of individual magnetic dipoles in a permanent (ferro)magnet
But what you might not expect is that in disordered, random materials — like amorphous solids or a series of randomly-oriented magnetic dipoles — their “memory” of what you do to them can last a very long time.
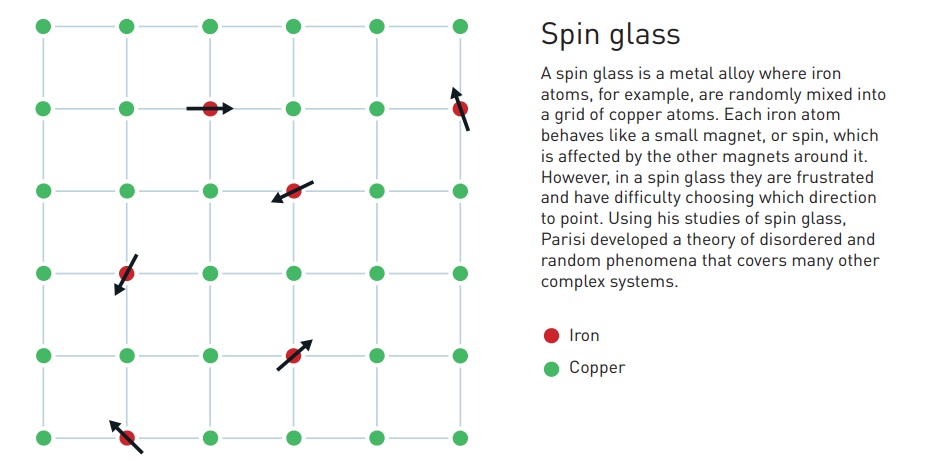
In analogy with the very first system we considered — where a system of arranged particles moves in a circle — imagine that the positions of every particle in your material are fixed, but they’re allowed to spin in whatever orientation they choose. The issue is this: Depending the spins of the adjacent particles, each particle will want to either align or anti-align with its neighbors, depending on which configuration yields the lowest-energy state.
But some configurations of particles — such as three of them in an equilateral triangle, where the only allowable spin directions are “up” and “down” — don’t have a unique, lowest-energy configuration that the system will tend toward. Instead, the material is what we call frustrated: It has to choose the “least worst” option available to it, which is very rarely the true lowest-energy state.
Combine disorder and the fact that these particles aren’t always arranged in a clean lattice, and a problem emerges. If you start your system off anywhere other than the lowest-energy state, it won’t return to equilibrium. Rather, it will reconfigure itself slowly and, for the most part, ineffectually: what physicist Steve Thomson calls “option paralysis.” It makes these materials incredibly difficult to study, and makes predictions about what configuration they’ll wind up in, as well as how they’ll get there, extraordinarily complex.
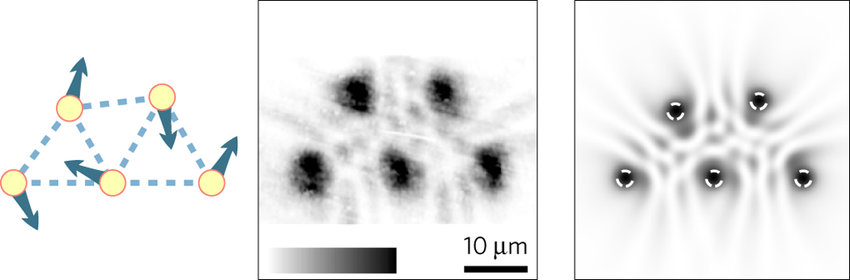
Just as Manabe and Hasselmann helped us get to that point for climate science, Parisi helped us get there for not just the specific materials known to exhibit these properties, i.e. spin glass, but also an enormous number of mathematically similar problems. The method first used to find an equilibrium solution to a solvable model of spin glass was pioneered by Parisi in 1979 with a then-novel method known as the replica method. Today, that method has applications ranging from neural networks and computer science to econophysics and other fields of study.
The most important takeaway from 2021’s Nobel Prize in physics is that there are incredibly complex systems out there — systems far too complex to make accurate predictions about simply by applying the laws of physics to the individual particles inside them. However, by modeling their behavior properly and leveraging a variety of powerful techniques, we can extract important predictions about how that system will behave, and we can even make quite general predictions for how changing the conditions in one particular fashion will alter the expected outcomes.
Congratulations to Manabe, Hasselmann, and Parisi, to the sub-fields of climate and atmospheric science and condensed matter systems, and to anyone who studies or works with complex, disordered, or variable physical systems. Only three individuals can win the Nobel Prize in any given year. But when humanity’s understanding of the world around us advances, we all win.