Ask Ethan: Where Does A Proton’s Mass Come From?

The whole should equal the sum of its parts, but doesn’t. Here’s why.
The whole is equal to the sum of its constituent parts. That’s how everything works, from galaxies to planets to cities to molecules to atoms. If you take all the components of any system and look at them individually, you can clearly see how they all fit together to add up to the entire system, with nothing missing and nothing left over. The total amount you have is equal to the amounts of all the different parts of it added together.
So why isn’t that the case for the proton? It’s made of three quarks, but if you add up the quark masses, they not only don’t equal the proton’s mass, they don’t come close. This is the puzzle that Barry Duffey wants us to address, asking:
What’s happening inside protons? Why does [its] mass so greatly exceed the combined masses of its constituent quarks and gluons?
In order to find out, we have to take a deep look inside.
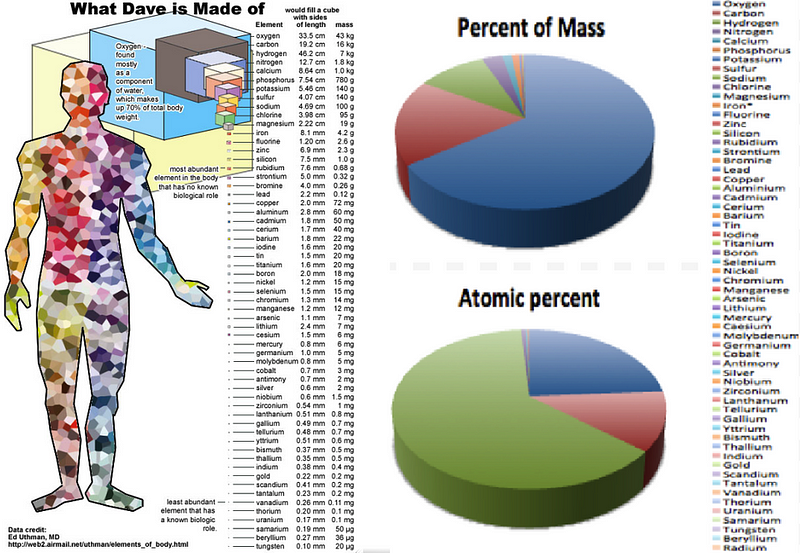
There’s a hint that comes just from looking at your own body. If you were to divide yourself up into smaller and smaller bits, you’d find — in terms of mass — the whole was equal to the sum of its parts. Your body’s bones, fat, muscles and organs sum up to an entire human being. Breaking those down further, into cells, still allows you to add them up and recover the same mass you have today.
Cells can be divided into organelles, organelles are composed of individual molecules, molecules are made of atoms; at each stage, the mass of the whole is no different than that of its parts. But when you break atoms into protons, neutrons and electrons, something interesting happens. At that level, there’s a tiny but noticeable discrepancy: the individual protons, neutrons and electrons are off by right around 1% from an entire human. The difference is real.
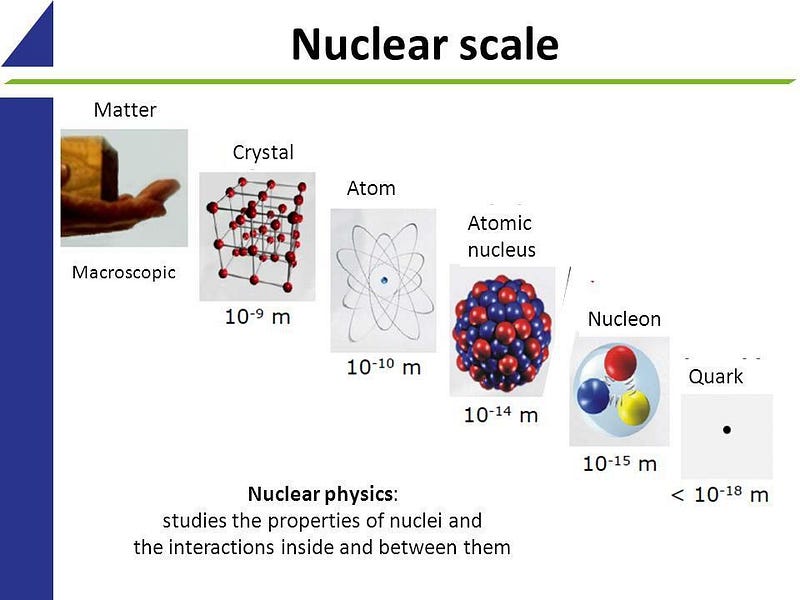
Like all known organisms, human beings are carbon-based life forms. Carbon atoms are made up of six protons and six neutrons, but if you look at the mass of a carbon atom, it’s approximately 0.8% lighter than the sum of the individual component particles that make it up. The culprit here is nuclear binding energy; when you have atomic nuclei bound together, their total mass is smaller than the mass of the protons and neutrons that comprise them.
The way carbon is formed is through the nuclear fusion of hydrogen into helium and then helium into carbon; the energy released is what powers most types of stars in both their normal and red giant phases. That “lost mass” is where the energy powering stars comes from, thanks to Einstein’s E = mc². As stars burn through their fuel, they produce more tightly-bound nuclei, releasing the energy difference as radiation.

This is how most types of binding energy work: the reason it’s harder to pull apart multiple things that are bound together is because they released energy when they were joined, and you have to put energy in to free them again. That’s why it’s such a puzzling fact that when you take a look at the particles that make up the proton — the up, up, and down quarks at the heart of them — their combined masses are only 0.2% of the mass of the proton as a whole. But the puzzle has a solution that’s rooted in the nature of the strong force itself.
The way quarks bind into protons is fundamentally different from all the other forces and interactions we know of. Instead of the force getting stronger when objects get closer, like the gravitational, electric, or magnetic forces, the attractive force goes down to zero when quarks get arbitrarily close. And instead of the force getting weaker when objects get farther away, the force pulling quarks back together gets stronger the farther away they get.
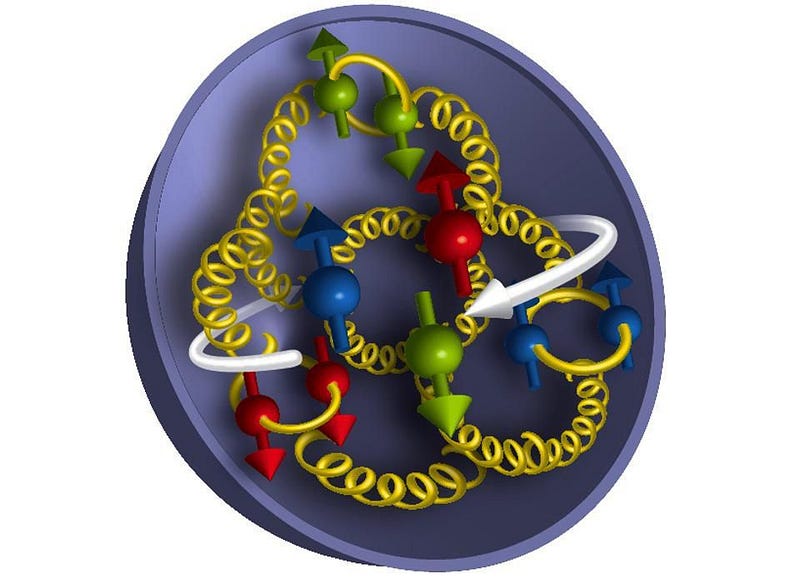
This property of the strong nuclear force is known as asymptotic freedom, and the particles that mediate this force are known as gluons. Somehow, the energy binding the proton together, responsible for the other 99.8% of the proton’s mass, comes from these gluons. The whole of matter, somehow, weighs much, much more than the sum of its parts.
This might sound like an impossibility at first, as the gluons themselves are massless particles. But you can think of the forces they give rise to as springs: asymptoting to zero when the springs are unstretched, but becoming very large the greater the amount of stretching. In fact, the amount of energy between two quarks whose distance gets too large can become so great that it’s as though additional quark/antiquark pairs exist inside the proton: sea quarks.

Those of you familiar with quantum field theory might have the urge to dismiss the gluons and the sea quarks as just being virtual particles: calculational tools used to arrive at the right result. But that’s not true at all, and we’ve demonstrated that with high-energy collisions between either two protons or a proton and another particle, like an electron or photon.
The collisions performed at the Large Hadron Collider at CERN are perhaps the greatest test of all for the internal structure of the proton. When two protons collide at these ultra-high energies, most of them simply pass by one another, failing to interact. But when two internal, point-like particles collide, we can reconstruct exactly what it was that smashed together by looking at the debris that comes out.

Under 10% of the collisions occur between two quarks; the overwhelming majority are gluon-gluon collisions, with quark-gluon collisions making up the remainder. Moreover, not every quark-quark collision in protons occurs between either up or down quarks; sometimes a heavier quark is involved.
Although it might make us uncomfortable, these experiments teach us an important lesson: the particles that we use to model the internal structure of protons are real. In fact, the discovery of the Higgs boson itself was only possible because of this, as the production of Higgs bosons is dominated by gluon-gluon collisions at the LHC. If all we had were the three valence quarks to rely on, we would have seen different rates of production of the Higgs than we did.

As always, though, there’s still plenty more to learn. We presently have a solid model of the average gluon density inside a proton, but if we want to know where the gluons are actually more likely to be located, that requires more experimental data, as well as better models to compare the data against. Recent advances by theorists Björn Schenke and Heikki Mäntysaari may be able to provide those much needed models. As Mäntysaari detailed:
It is very accurately known how large the average gluon density is inside a proton. What is not known is exactly where the gluons are located inside the proton. We model the gluons as located around the three [valence] quarks. Then we control the amount of fluctuations represented in the model by setting how large the gluon clouds are, and how far apart they are from each other. […] The more fluctuations we have, the more likely this process [producing a J/ψ meson] is to happen.

The combination of this new theoretical model and the ever-improving LHC data will better enable scientists to understand the internal, fundamental structure of protons, neutrons and nuclei in general, and hence to understand where the mass of the known objects in the Universe comes from. From an experimental point of view, the greatest boon would be a next-generation electron-ion collider, which would enable us to perform deep inelastic scattering experiments to reveal the internal makeup of these particles as never before.
But there’s another theoretical approach that can take us even farther into the realm of understanding where the proton’s mass comes from: Lattice QCD.

The difficult part with the quantum field theory that describes the strong force — quantum chromodynamics (QCD) — is that the standard approach we take to doing calculations is no good. Typically, we’d look at the effects of particle couplings: the charged quarks exchange a gluon and that mediates the force. They could exchange gluons in a way that creates a particle-antiparticle pair or an additional gluon, and that should be a correction to a simple one-gluon exchange. They could create additional pairs or gluons, which would be higher-order corrections.
We call this approach taking a perturbative expansion in quantum field theory, with the idea that calculating higher and higher-order contributions will give us a more accurate result.
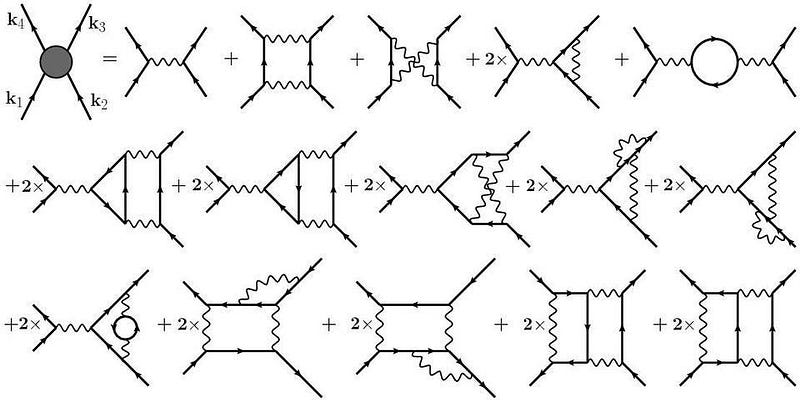
But this approach, which works so well for quantum electrodynamics (QED), fails spectacularly for QCD. The strong force works differently, and so these corrections get very large very quickly. Adding more terms, instead of converging towards the correct answer, diverges and takes you away from it. Fortunately, there is another way to approach the problem: non-perturbatively, using a technique called Lattice QCD.
By treating space and time as a grid (or lattice of points) rather than a continuum, where the lattice is arbitrarily large and the spacing is arbitrarily small, you overcome this problem in a clever way. Whereas in standard, perturbative QCD, the continuous nature of space means that you lose the ability to calculate interaction strengths at small distances, the lattice approach means there’s a cutoff at the size of the lattice spacing. Quarks exist at the intersections of grid lines; gluons exist along the links connecting grid points.
As your computing power increases, you can make the lattice spacing smaller, which improves your calculational accuracy. Over the past three decades, this technique has led to an explosion of solid predictions, including the masses of light nuclei and the reaction rates of fusion under specific temperature and energy conditions. The mass of the proton, from first principles, can now be theoretically predicted to within 2%.

It’s true that the individual quarks, whose masses are determined by their coupling to the Higgs boson, cannot even account for 1% of the mass of the proton. Rather, it’s the strong force, described by the interactions between quarks and the gluons that mediate them, that are responsible for practically all of it.
The strong nuclear force is the most powerful interaction in the entire known Universe. When you go inside a particle like the proton, it’s so powerful that it — not the mass of the proton’s constituent particles — is primarily responsible for the total energy (and therefore mass) of the normal matter in our Universe. Quarks may be point-like, but the proton is huge by comparison: 8.4 × 10^-16 m in diameter. Confining its component particles, which the binding energy of the strong force does, is what’s responsible for 99.8% of the proton’s mass.
Send in your Ask Ethan questions to startswithabang at gmail dot com!
Ethan Siegel is the author of Beyond the Galaxy and Treknology. You can pre-order his third book, currently in development: the Encyclopaedia Cosmologica.