Ask Ethan: Can Gravitational Waves Pass Through Black Holes?
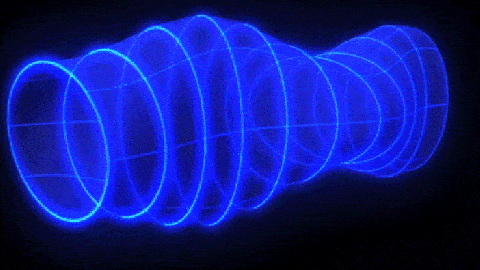
And either way, is energy or information conserved?
When two things in the Universe that “always” occur meet one another, how do you know which one will win? Gravitational waves, for example, always pass through whatever they encounter: empty space, dark matter, gas clouds, plasma, dust, planets, stars, and even dense stellar remnants like white dwarfs and neutron stars. They carry energy, which they can deposit into objects they affect, deforming and distorting space (along with everything in it) as they pass through. Nothing ever seems to stop gravitational waves, with the only alterations we see coming from the effects of distorted spacetime due to the presence of masses and the expanding Universe.
But on the other side of the coin, we have black holes, which have an event horizon: a region from within which nothing can escape. So when the immovable object meets the irresistible force, who wins? That’s what Rhys Taylor wants to know, asking:
“There’s plenty on the internet (including your own pieces) about how gravitational waves do not really escape from the event horizon, but this appears to always be about gravitational waves emitted by the black hole itself: e.g., during a merger… what happens to a gravitational wave produced by some distant external event?”
Would it just pass through the black hole itself? Or would it somehow get absorbed? It’s a fascinating question to explore.
Let’s start with black holes: objects that are not to be trifled with in the Universe. When you’re far away from a black hole’s event horizon, it appears to behave just like any other ordinary mass in the Universe. From the location of Earth, for example, the gravitational effects that we experience from our Sun are indistinguishable from the ones that would be generated by:
- a white dwarf,
- a neutron star,
- or a black hole,
- of the same exact mass.
We would still experience the same orbit, with the same speed, the same period, and the same elliptical pattern (and even the same level of relativitistic precession) that we experience from our Sun. The only differences that would be perceptible would appear when we looked in the vicinity of the Sun (or whatever replaced it) itself. The bending of background starlight, along with all other forms of matter and radiation, is intensified the closer and closer you get to a compact, massive object: regions that are currently obscured by the disk of the Sun. Other than the distortion of space from the innermost ~1 degree closest to the center of the Sun, where the curvature of space is most severe, there are no other detectable differences.
But that inner region of space matters tremendously when we consider the impact it has on absorbing various types of matter and radiation. For example:
- the Sun, being an opaque object, would absorb everything that it interacts with, like protons, neutrons, electrons, and photons, but would be transparent to particles like neutrinos and antineutrinos,
- white dwarfs, being opaque but much smaller than the Sun, would have a much smaller cross-sectional area (perhaps only ~0.01% that of the Sun) but would still be opaque to protons, neutrons, electrons, and photons, and due to its density would begin absorbing a small fraction of the neutrinos that strike it,
- neutron stars, even smaller and denser than white dwarfs, have a much lower area over which they absorb protons, neutrons, electron, and photons, but will absorb ~100% of the ones that strike it, along with up to ~50% of the neutrinos (and antineutrinos) that pass through its diameter,
- and black holes absorb absolutely 100% of everything we know of that touches or crosses its event horizon.
From a black hole, if you’re an energy-carrying entity, there ought to be no escape.
What does all of this mean for gravitational waves, though? Unlike every other quantum of matter or radiation, gravitational waves aren’t typically thought of as particles that propagate through spacetime, but rather as a form of radiation that is itself a ripple in the fabric of spacetime. When a gravitational wave passes through a region of space that contains matter or energy, everything in that region also experiences the same distortions — the same compressions and rarefactions — that the space it’s occupying experiences.
The important factor that we have to consider, though, is what happens to the matter that exists in the space that a gravitational wave passes through? Yes, as the waves pass through us, they shorten and lengthen the distances between every quantum of matter that exists. But, can these waves deposit energy into the matter that they interact with? Believe it or not, that was the main subject of an intense conference in 1957 dubbed GR1: the first American conference on General Relativity.
The argument that wound up deciding the issue was put forth by Richard Feynman, and today it’s known as the sticky bead argument. Imagine, as in the image above, that you have two thin, perpendicular rods, each with beads on the end. On each rod, one bead is fixed: it is attached to the rod and cannot move. But the other bead is free to slide; if a gravitational wave passes through the rod perpendicular to the rod’s direction, the distance between the beads will now change.
If the bead and rod are frictionless, there’s no heat produced and no energy “taken” from the gravitational waves; that motion comes free-of-charge. But as soon as you introduce friction, the motion of the bead against the rod causes the atoms/molecules/electrons to rub against one another, producing heat through friction, and thereby extracting energy from the gravitational waves. Feynman’s argument doesn’t merely demonstrate that gravitational waves carry energy, but shows how to extract that energy from the waves and put it into a real, physical system.
This is precisely the principle that modern-day gravitational wave detectors rely on to reconstruct the gravitational wave signals that pass through their enormous, perpendicular laser arms. When these gravitational waves pass through our planet, everything that’s on our planet absorbs the correspondingly relevant amount of energy from the waves owing to the changes experienced in the positions and interactions of the particles we have. In the case of LIGO, above, this led us to not only detect gravitational waves, but to measure their properties and infer the total amount of energy created in the events that first gave rise to them.
Observationally, there isn’t that much direct evidence for the properties of gravitational waves, however. We can look at the orbits of binary pulsars, for example, and conclude how much energy is being radiated away in the form of gravitational waves, and get a prediction that matches up extremely well with the observed orbital changes of that binary pulsar system.
We also have about ~60 total observations of merging compact objects from LIGO and Virgo, including one multi-messenger event: where gravitational waves and electromagnetic radiation were detected in short succession from one another, emanating from the same source. Although that’s just one out of 60 — and it’s probably important to note that the only other neutron star-neutron star merger we’ve seen didn’t have an observed electromagnetic counterpart — it did teach us some incredibly important information.
We learned that:
- gravitational waves and electromagnetic waves travel at the same speed, the speed of light, to within 1-part-in-10¹⁵,
- that electromagnetic waves are slowed by their passage through matter, while gravitational waves are not,
- that both electromagnetic and gravitational waves have their wavelength stretched by the expansion of the Universe,
- and that gravitational lensing and gravitational redshift affect both photons and gravitational waves in the same exact fashion.
In other words, when gravitational waves travel through the Universe, though, they do experience the same effects that photons do owing to General Relativity.
So now, let’s put some pieces together. Gravitational waves carry energy, and are predicted to behave — in the context of General Relativity — the same way that photons do in a whole bunch of ways. They both:
- experience relativistic redshifts/blueshifts dependent on the strength of the gravitational field, the curvature of space, and the relative motions of the source and observer,
- have their propagation direction deflected by the presence of massive objects,
- experience identical gravitational lensing effects,
- carry energy and experience a change in that energy owing to the expansion of the Universe,
- and can deposit energy (or not) into objects that they pass through/into, depending on the strength/coupling of the interaction.
The biggest differences, on the other hand, are only twofold. One is that these waves have a tensor-like quality rather than simply a vector-like quality; they are a fundamentally different type of radiation. And the other is that the quantum counterpart of electromagnetic radiation, the (spin=1) photon, is known to exist and has had its properties measured. The quantum counterpart of gravitational radiation, the (spin=2) graviton, is only theorized; it has never been measured or detected directly.
However, regardless of those differences, the fact that gravitational waves follow the null geodesics of curved space give us one unambiguous answer to the original question: when an external gravitational wave propagates into a region of space where there is an event horizon, what happens to those waves?
The answer is straightforward: they propagate in the same fashion that any massless quanta would travel, following the path laid out by the curved space that they propagate through. If that path takes you close to the event horizon of a black hole, you’ll experience all the “normal” relativistic phenomena (redshift/blueshift, time dilation/length contraction, frame-dragging, etc.), but you’ll still be able to escape so long as you don’t cross the event horizon.
If you do cross it, however, there’s only one option: you fall inexorably towards the central singularity, and upon crossing over the threshold of the event horizon, your energy and your angular momentum — both of which gravitational waves should possess with respect to the black hole — get added to the black hole itself. In other words, black holes do grow from devouring all they encounter, and gravitational waves help that to occur.
Despite the fact that gravitational waves are ubiquitous and are generated all throughout the galaxy and the Universe, the reality is that the cross-sectional area of a black hole’s event horizon is so minuscule, even for the largest of all black holes, that the amount of energy added from the absorption of gravitational waves is completely negligible. The infall of normal matter, dark matter, neutrinos, and even regular (electromagnetic) radiation vastly outstrips the energy gain from incoming gravitational radiation. When all is said and done, there just isn’t enough of it in the Universe to make a substantial change to the total amount of mass/energy in a black hole.
But it happens. The ripples of the gravitational waves — just like anything else that falls into a black hole — must get imprinted onto the surface of the black hole, conserving information, while the energy and angular momentum get absorbed into the black hole, conserving those quantities as well. Every time one of these “ripples in spacetime” passes across a black hole, a small fraction of its energy gets absorbed. It’s tiny, because gravitational waves spread out in a sphere from the source and only a tiny “disk” proportional to the event horizon’s area acts to absorb it, but any non-zero effect still counts. May the day come where we’re actually savvy enough to measure it!
Send in your Ask Ethan questions to startswithabang at gmail dot com!
Starts With A Bang is written by Ethan Siegel, Ph.D., author of Beyond The Galaxy, and Treknology: The Science of Star Trek from Tricorders to Warp Drive.