Ask Ethan: Can We Find Out If Gravitons Exist?
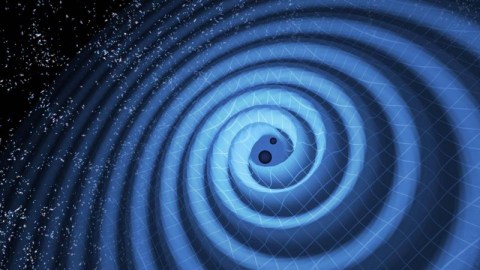
They’re not just a theoretical prediction of quantum gravity. They should be detectable, too.
The Universe, if you look at it closely and carefully enough, is fundamentally quantum in nature. If you try and divide matter up into smaller and smaller pieces, eventually you arrive at indivisible components that cannot be broken up any further. These particles interact by exchanging a specific type of quantum that couples to their various charges. Gluons mediate the strong nuclear force, interacting with particles that have a color charge. The W and Z bosons mediate the weak force, coupling to the particles that have weak hypercharge and isospins. And the photon mediates the electromagnetic force, acting on particles with an electric charge. Gravitation, though, might be the outlier. Our theory of gravitation is classical: General Relativity. In theory, though, there should be a quantum counterpart, mediated by a hypothetical quantum particle known as the graviton. Only, is it possible to find out whether gravitons actually exist? That’s what Mark Richards wants to know, asking:
“Is there a physics case for the existence of gravitons, or are they just needed to satisfy quantum gravity? […] What are your own thoughts on gravitons?”
We don’t know, experimentally or observationally, whether gravity is inherently quantum in nature or not. If it is, we’ll accept it as soon as there’s a way to test and verify it. Here’s why that might not be as impossible as you might initially think.
Arguably, the first quantum particle ever discovered was the photon: the quantum associated with light. While it’s true that photons mediate the electromagnetic force, the photons that do so are virtual: they provide us with a way of calculating the electromagnetic field that permeates all of space. That stands in contrast to real photons: the photons we can emit, absorb, and otherwise measure in our instruments and detectors.
Every time you see something, that’s a result of a photon exciting a molecule in the rods or cones present in the retinas of your eyes, which then stimulates an electrical signal to your brain, which interprets the set of data coming in and constructs an image of what you observed. The act of seeing is an inherently quantum act, with each photon carrying a specific amount of energy that either will or won’t be absorbed by particular molecules. Although the photoelectric effect, first described by Einstein, was what demonstrated the quantum nature of light, it’s important to recognize that all light is quantum in nature.
We can describe many of the phenomena associated with light perfectly well by viewing light as a wave, however, and gravitation has what’s quickly become a well-known analogue: gravitational waves. Just as a charged particle moving through an electromagnetic field will emit electromagnetic waves (in the form of photons), a mass moving through a region of curved spacetime (which is the analogue of a gravitational field) will emit gravitational radiation, or gravitational waves.
When the advanced LIGO detectors began taking data in 2015, they quickly began discovering the strongest sources of gravitational radiation in the Universe in the frequency range that the interferometers were sensitive to: merging black holes. Over the past 5 years, those detectors were upgraded, joined by the Virgo detector, and have to date discovered more than 50 total gravitational wave events. From merging black holes to merging neutron stars to, quite possibly, neutron stars merging with black holes, they’ve demonstrated that gravitational radiation is very real, and in agreement with Einstein’s predictions.
The big question then becomes, once we know gravitational waves are real, whether they exhibit wave-particle duality as well? In other words, just as photons exhibit wave-like properties but also particle-like, quantum properties, is the same thing true for gravitational waves? Is there a particle-like counterpart that this radiation is made of, with the tremendous amounts of energy carried by gravitational waves distributed into individual, discrete quanta?
It’s a compelling and eminently reasonable idea. Water waves, for example, are made of particles, even though they don’t appear that way. But if you were to float, say, a bunch of ping pong balls atop the surface of the water, you can get an idea for visualizing what’s truly occurring. Individual ping pong balls would move up-and-down, back-and-forth, etc., along the surface of the water, and you can imagine that the individual molecules along a wavy surface of water are doing something similar. As Mark — our question-asker for this week — correctly surmised, the quantum counterparts of gravitational waves, gravitons, are fully expected to emerge if gravity is fundamentally quantum in nature.
Even though there’s an awful lot we don’t yet know about gravitational waves, including whether they’re made of individual quanta or not, there are a lot of properties we have been able to discern. Some of the more interesting ones are as follows:
- gravitational waves do carry real, finite, measurable amounts of energy that can be deposited into detectors,
- gravitational waves propagate at a specific speed through space, specifically, the speed of gravity, which differs from the speed of light by no more than ~1 part in 10¹⁵,
- gravitational waves compress-and-expand the space they travel through in mutually perpendicular directions, which enables a clever setup (such as those employed by LIGO and Virgo) to detect them,
- and they ought to interfere with any other ripples in space both constructively and destructively, obeying the same rules that any other wave would obey.
In addition, we’ve already observed that gravitational waves, just like photons, do indeed stretch their wavelengths as they travel through the expanding Universe. As the background of the underlying space expands, so do the wavelengths of the gravitational waves we observe.
But all of this would be true whether gravitation were purely classical in nature or whether there were a more fundamental quantum theory of gravity that Einstein’s General Relativity is only an approximation for. If it’s quantum, that implies that every gravitational wave we see, in analogy with every light wave that we see:
- is made of a large number of quantum particles,
- where each quantum has an inherently zero rest mass,
- meaning that it propagates at the speed of light (which equals the speed of gravity).
In addition, there are a few properties that would be unique to gravitons: properties that it wouldn’t share with photons. One of them is that, owing to the nature of the theory of gravitation, the particle that mediates the gravitational force would have to have a spin of 2, rather than a spin of 1 like the photon. Because it’s massless, its spin can only be +2 or -2; it can have no intermediate value. Additionally, gravitons would only interact through the gravitational force. They’d respond to any other quantum that had mass or carried energy, but they should be uncharged (and hence, would be unaffected) under all of the other fundamental interactions.
One way that the Universe could surprise us would be if gravitons turned out to actually have a very tiny, non-zero rest mass. Just as many of the fundamental particles (even including some of the force-carrying bosons, such as the W-and-Z bosons from the weak interactions) have a finite mass inherent to them, it’s possible that a graviton might as well. From our current gravitational wave measurements, however, and the energy received by our detectors, we’ve constrained the graviton’s mass to be mind-bogglingly tiny. If it does have a mass, it’s got to be less than 1.6 × 10^-22 eV/c², or some ~10²⁸ times lighter than the electron.
You might be inclined to brute-force your way into detecting a graviton: by building a particle accelerator that was powerful enough to start producing measurable amounts of them, for example. In theory, there’s no reason why we couldn’t do this, as the energy your (circular) particle accelerator achieves is simply related to the ring’s radius and the bending strength of the magnets. With cutting-edge magnet technology, the same sized ring that we could use to test String Theory — about the size of Pluto’s orbit in the Solar System — would also probe the existence of gravitons.
That doesn’t seem very likely, nor does the next brute force option: simply build a large enough, sensitive enough detector to detect whatever gravitons would be naturally produced by other astrophysical phenomena in the Universe. Sabine Hossenfelder estimates “we would need a detector the size of planet Jupiter to measure a graviton produced elsewhere,” something that’s unlikely to happen anytime soon.
The key place to look for gravitons — or a signature of the “particle” part of the nature of these gravitational waves that we’ve demonstrated exist — would be where quantum gravitational effects are anticipated to be strongest and most pronounced: at the shortest distance scales and where gravitational fields are strongest. There’s no better place in the Universe to probe this regime than where two black holes merge, as close to their singularities as you can conceivably get.
General Relativity is perfectly adequate, for all the black holes expected to exist in our Universe, for describing the entirety of the effects that happen outside of a black hole’s event horizon. But when you get very close to a singularity, or specifically when two singularities merge together to create a different singularity, we anticipate that quantum effects may show up: quantum effects that signal a departure from the predictions of General Relativity. If ever there were a place where phenomena inherent to quantum gravity would show up, that would be it.
If we wanted to realistically do that, we’d have to be able to take data right around the exact moment the singularities merged, and we’d have to do it on extremely fast timescales. Today, LIGO is sensitive to events that occur on ~millisecond timescales, but if we could probe the Universe on sub-picosecond timescales — including at the very end of the inspiral phase, at the moment of the merger, and at the start of the subsequent ringdown phase — that might be possible. We presently have laser pulses that hit the femtosecond or even attosecond timescales (10^-15 s to 10^-18 s), and with enough interferometers working at once, we might be sensitive enough to actually detect any signatures of quantum gravity.
The bigger problem, though, is this: most of the signatures that we can envision detecting that would reveal whether gravitation is quantum in nature wouldn’t directly reveal the existence of gravitons. Detecting the much sought-after B-modes predicted by cosmic inflation would indirectly demonstrate that gravitation is inherently quantum in nature, but there would be no direct detection of gravitons. If you fired an electron through a double slit and could measure whether its gravitational field passed through both slits or just one, that would reveal whether gravity was quantum in nature or not, but again, we wouldn’t detect gravitons.
Other schemes exist as well, and they’re very clever. If you passed photons of various wavelengths through a crystal and the “steps” the crystal moved were discrete instead of continuous, you could prove that space was quantized. If you brought masses into a quantum superposition of states and the energy levels were dependent on gravitational self-energy, you could determine whether gravity was quantized or not. And there are other potential signatures as well that could indirectly reveal whether gravity is inherently quantum in nature.
The possibility is exciting and tantalizing, but we have to remember that taking the first step looks very different from drawing the ultimate goal-of-a-conclusion we’re all truly looking forward to. If we could demonstrate that gravity is inherently quantum in nature, that would be tremendous. If we could demonstrate that space is quantized, that would change how we view our reality. And if we could perform an experiment whose results disagreed with the straightforward predictions of General Relativity, that would spur us towards tremendous developments and new advances.
But none of that would be the same as demonstrating that gravitons actually exist, anymore than measuring the orbital decay of pulsing neutron stars demonstrated that gravitational waves really exist. Yes, that discovery was a tremendous, Nobel-winning achievement, and it was consistent with everything we now think about gravitational waves. But it didn’t prove that gravitational waves existed; we needed direct detection for that. For right now, our next step should be to perform an experiment that indicates General Relativity isn’t enough, and reveals a hint of the Universe’s theorized quantum gravitational nature. The dream of directly detecting gravitons is a much larger prize: one that we expect to be far more impractically difficult to actually achieve.
Send in your Ask Ethan questions to startswithabang at gmail dot com!
Starts With A Bang is written by Ethan Siegel, Ph.D., author of Beyond The Galaxy, and Treknology: The Science of Star Trek from Tricorders to Warp Drive.