Lithium Mystery Solved: It’s Exploding Stars, Not The Big Bang Or Cosmic Rays
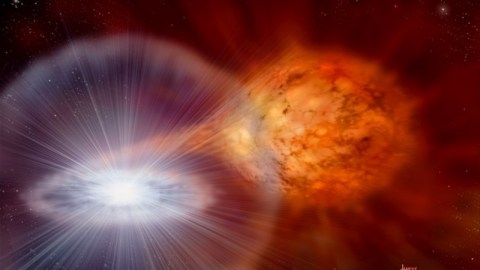
The origin of the 3rd element on the periodic table was one of the great cosmic mysteries. We just solved it.
How did we form the elements that pervade the Universe today? They come from a variety of sources. Some were formed over 13 billion years ago, in the earliest stages of the hot Big Bang. Others weren’t formed until much later, forged in stars and various astrophysical cataclysms. Still others come from particle collisions in space: where high-energy cosmic rays run into atomic nuclei, splitting them apart into rare, light elements.
Of all the elements on the periodic table, one of the hardest to account for is lithium: the third element of all. We observe that it exists on Earth, throughout the Solar System, and all over the galaxy but we’ve been unable to explain how it’s made. However, new research led by astrophysicist Sumner Starrfield has just solved the puzzle, finding precisely the right amount that was missing. The culprit? An often-overlooked class of exploding stars: classical novae. Here’s what we’ve learned.
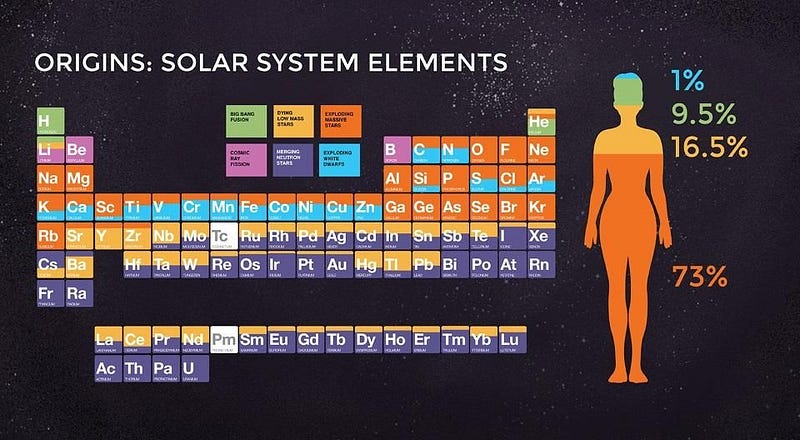
If you want to explain how anything in the Universe came to be, there are three steps you have to take.
- First, you have to measure how much of the “stuff” you’re trying to measure is actually out there.
- Second, you have to understand the theoretical physics that drives the different types of ways to produce the “stuff” you’ve encountered.
- And finally, you have to measure the events themselves that drive the production of this “stuff,” and put all the pieces together.
For approximately 60 years, lithium has been a puzzle where the pieces all failed to add up. We have three different ways that we know of to make lithium: from the Big Bang, from cosmic rays slamming into heavier atomic nuclei and splitting them apart, and from a very delicate process that occurs in stars under only very specific conditions. Yet, when we add up all the different ways that we knew of to make this lithium, they couldn’t even account for 20% of the total. Here’s where the mismatch came from.
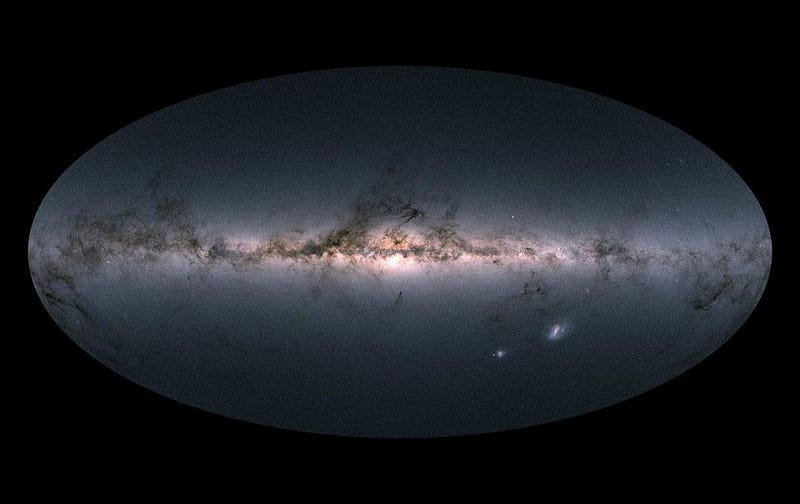
If you want to know how much lithium is out there in the galaxy, you must arrive at some way to measure it. With some 400 billion stars in our galaxy, we’ve measured enough of them — their masses, radii, color, temperature, abundances of heavy elements, etc. — to know how they compare to our own Sun. By measuring how much lithium is in our own Solar System, and understanding how our Solar System fits into the larger context of our galaxy, we can arrive at a very good estimate for how much lithium is found throughout the entire galaxy.
Lithium is extremely fragile, with just three protons in its nucleus and a very loosely-held outer electron, so it’s easy to destroy in stars and very easy to ionize (and, therefore, to miss) when we look for it astronomically. But it’s preserved in asteroids and comets: the pristine material that formed our Solar System in its earliest stages. From the meteorites we’ve examined, we can reconstruct exactly how much lithium is found throughout the entire galaxy: about 1,000 solar masses worth.
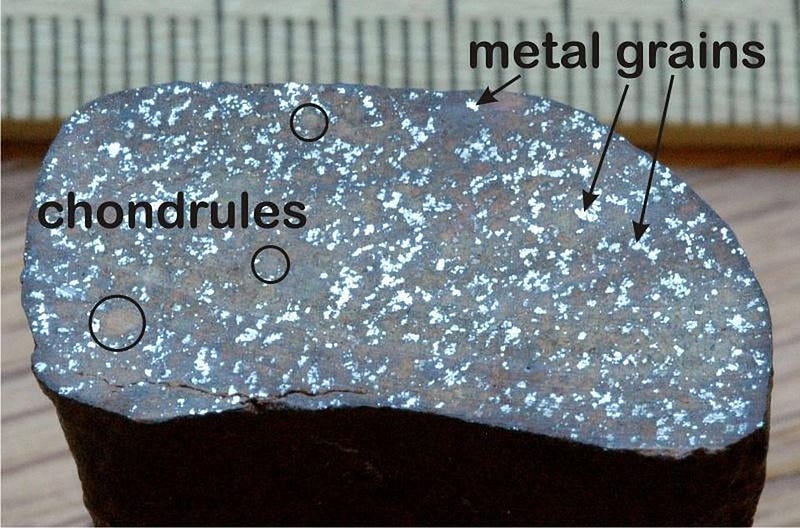
So if that’s how much lithium we have, how did we make it?
In the early stages of the hot Big Bang, things were so energetic and so dense that nuclear fusion spontaneously occurred among the primordial protons and neutrons, producing a large amount of the lightest elements. By the time the Universe is about 4 minutes old, a sea of raw protons and neutrons has been converted into:
- 75% hydrogen (including deuterium and tritium),
- 25% helium (including helium-3 and helium-4),
- and about 0.00000007% beryllium-7, produced in tiny amounts.
With a half-life of 53 days, that beryllium-7 will capture an electron and decay into lithium-7, which is stable. It isn’t until millions of years later, when stars begin forming, that any heavier elements are formed. From this leftover lithium-7, dating all the way back to the Big Bang, we should have about 80 solar masses worth of lithium in our galaxy: only about 8% of what’s out there.
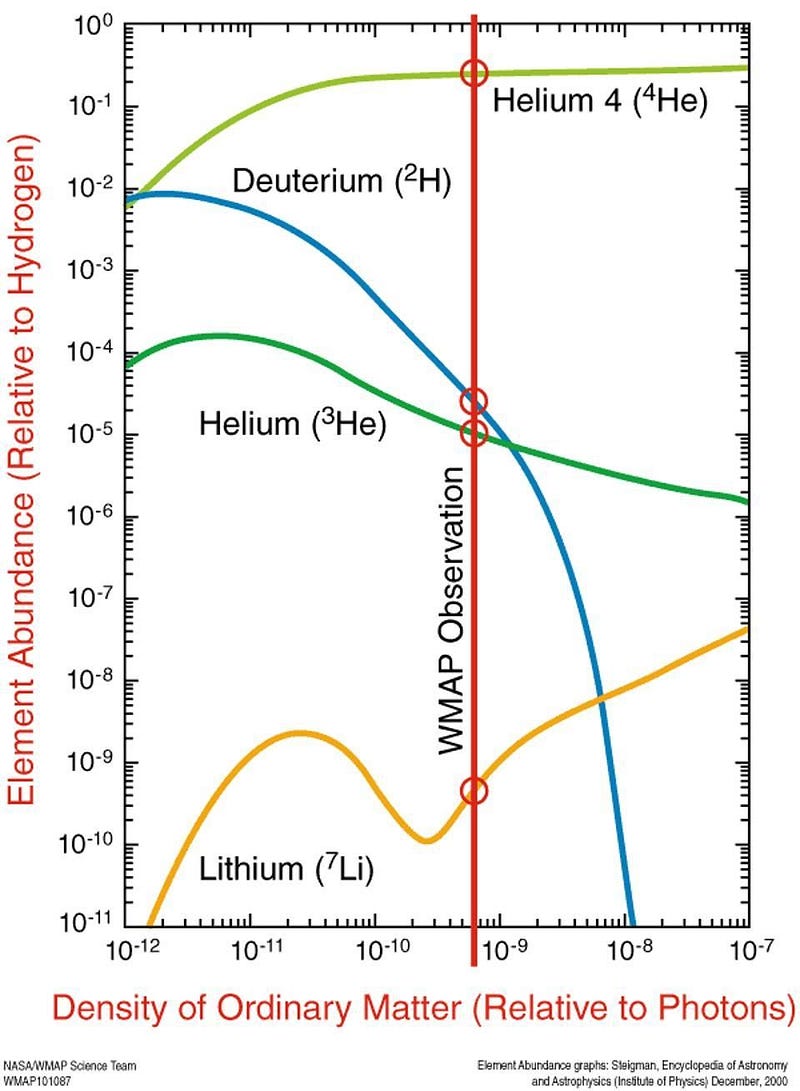
There’s another way to make lithium: from what’s known as cosmic ray spallation. Stars, pulsars, white dwarfs, black holes, and many other astrophysical sources emit high-energy particles known as cosmic rays, which fly through the Universe at speeds so fast they’re practically indistinguishable from the speed of light. When they collide with heavy elements — the elements produced in stars — they can blast them apart into smithereens.
Those smithereens include three of the lightest elements: lithium (element #3), beryllium (element #4), and boron (element #5). Because stars fuse hydrogen into helium and then go straight from helium into carbon, these three elements aren’t produced in most stars, and instead need this spallation process to create them. This is where practically all of the lithium-6 (with three neutrons) comes from, but it produces only a negligible amount of lithium-7: the majority of lithium found in the galaxy. This route, too, is no good.
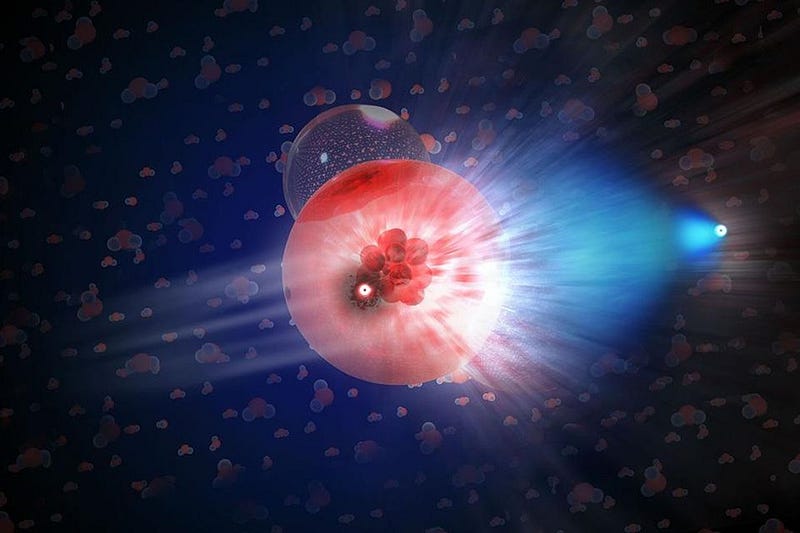
So it’s got to be the other option: there’s got to be some way of making this missing lithium-7 in stars. For a long time, going all the way back to the time of Fred Hoyle some 60 years ago, we’ve known of a way to do it: in red giant stars undergoing a particular stage in their lives. You can’t make the lithium itself (because it’s too fragile), but just like the Big Bang, you can create beryllium-7 in the cores of these giant stars.
If the material remained in the core, it would decay into lithium and then be destroyed by the high-energy conditions found there. But the saving grace is that red giant stars can go through phases where they convect: dredge-up phases, which transports material from the core to the cooler, sparser outer layers. When these stars then die, the lithium-7, now found in the outer layers, gets blown off and returned to the interstellar medium.
This actually does produce lithium, and more lithium than the Big Bang makes: about 100 solar masses worth when you add up what’s expected over the entire galaxy. But this is only about 10% of what we need: the other ~800+ solar masses are unaccounted for. There was one other major idea that persisted for how lithium might be formed in the Universe, but the technology didn’t exist to make the necessary measurements until the last few years.
The possible culprit? A very old class of stellar cataclysms known as classical novae. When stars like our Sun die, they leave a stellar remnant behind known as a white dwarf: a core of dense atoms typically comprised of carbon and oxygen atoms. Many stars are like our Sun, but not every Sun-like star is in a system is like our own; many of them have binary companions. And when a normal or giant star orbits a white dwarf, the denser white dwarf can start siphoning that loosely-held matter off of its companion star.
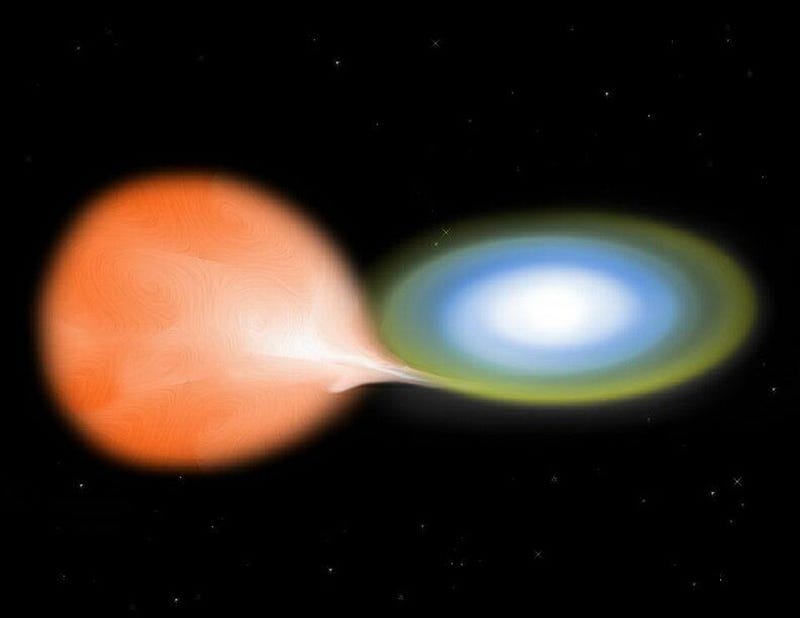
Over time, white dwarfs can steal enough matter that nuclear fusion ignites: right at the interface of the carbon-and-oxygen atoms with the material accrued from the neighboring star. A runaway reaction occurs, producing a variety of elements — including, in theory, beryllium-7 — and then all of those atoms are ejected back into the interstellar medium. We’ve been measuring novae for centuries, but didn’t have the instruments necessary to check for beryllium-7 or lithium-7 until the last few years.
But all of that has changed. Teams of scientists using both the Subaru telescope and the Very Large Telescope were finally able to detect and measure beryllium-7 from these classical novae, while Starrfield’s team used the Large Binocular Telescope to measure the presence of lithium-7 directly in the afterglow of these novae. Spectacularly, when we calculate the estimated abundances, it’s greater than the amount produced in red giant stars: and possibly even enough to account for the amount that’s been missing for so long.
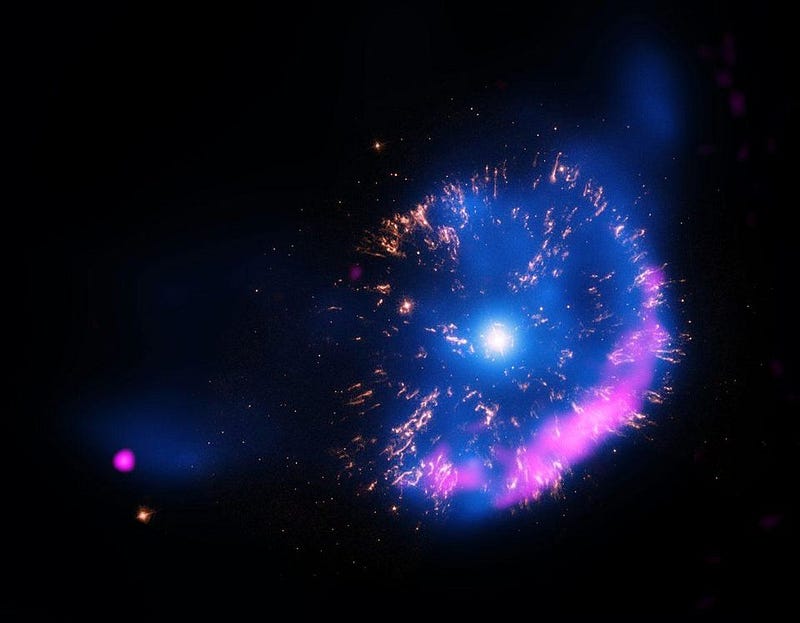
This is a spectacular result that answers the longstanding puzzle of where our Universe’s lithium most likely comes from: it primarily originates from classical novae. We also learned based on what was seen ejected from these novae and how quickly that material from the white dwarf’s core has to mix with the accreted matter, but only during the detonation itself, not before. It’s a definitive conclusion to one of the most longstanding questions in astrophysics: the origin of element #3 on the periodic table.
Like almost all discoveries in science, however, this one raises a bunch of new questions that now drive the field forward. They include:
- Do oxygen-neon white dwarfs also produce lithium, or only carbon-oxygen white dwarfs?
- Do all carbon-oxygen white dwarfs that experience novae produce lithium, or only some of them?
- Are lithium-7, produced from novae, and lithium-6, produced from cosmic ray spallation, actually correlated?
- And, if we can improve the precision of our measurements, do theory and observation actually line up exactly? Or will there still be a mismatch, after all?
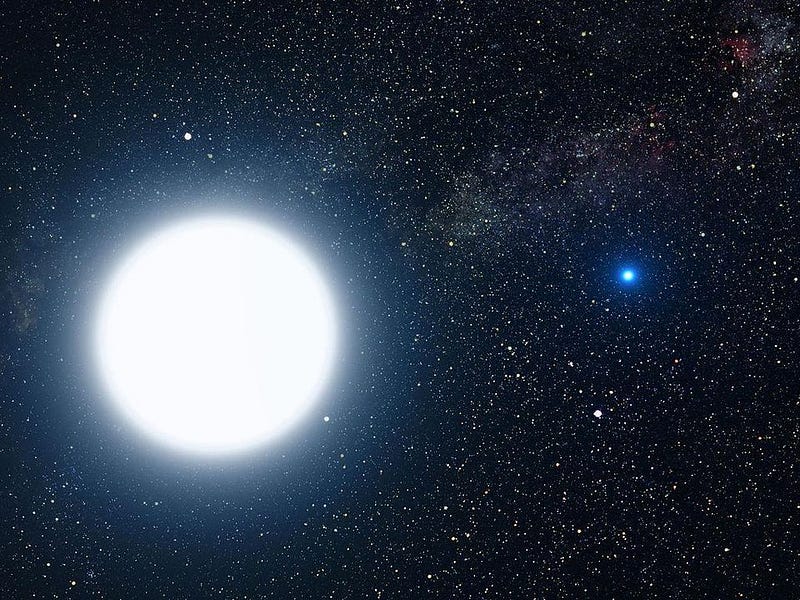
After more than half a century of not understanding where the lithium we see in our Universe comes from, astronomy has finally yielded the answer: from the classical novae occurring throughout the galaxy and beyond. Matter from a companion star get siphoned onto a white dwarf, and when a critical threshold is crossed, a fusion reaction — involving the accrued matter as well as material from the white dwarf itself — creates beryllium-7, which then decays to make our Universe’s lithium.
In the coming years, NASA’s infrared James Webb Space Telescope and the wide-field Nancy Roman Telescope will team up to find and measure not just a handful of these novae, but likely hundreds of them. For the Universe, making the first two elements is easy, as is making carbon and the heavier elements. But lithium, for astronomers, has been a mystery since we first discovered it. At last, the puzzle has finally been solved.
The author thanks Sumner Starrfield for an incredibly useful discussion concerning classical novae and cosmic lithium.
Ethan Siegel is the author of Beyond the Galaxy and Treknology. You can pre-order his third book, currently in development: the Encyclopaedia Cosmologica.