Ask Ethan: What Does ‘Truth’ Mean To A Scientist?

It’s very different from the colloquial meanings of “true-and-false” or “right-and-wrong.”
In many ways, the human endeavor of science is the ultimate pursuit of truth. By asking the natural world and Universe questions about itself, we seek to gain an understanding of what the Universe is like, what the rules that govern it are, and how things came to be the way they are today. Science is the full suite of knowledge that we gain from observing, measuring, and performing experiments that test the Universe, but it’s also the process through which we perform those investigations. It might be easy to see how we gain knowledge from that endeavor, but how do scientists arrive at the idea of a scientific truth? That’s Curtis Brand’s question, as he asks:
I was speaking to a friend [who’s] an economic analyst, and his personal definition of a truth was when something’s 51%+ likely to happen… In science, do you ever truly accept anything as a truth, and if so, on what grounds do you typically decide its worthy of being called “true”?
When we’re speaking scientifically, “truth” is something very different than how we colloquially use it. Here’s how.
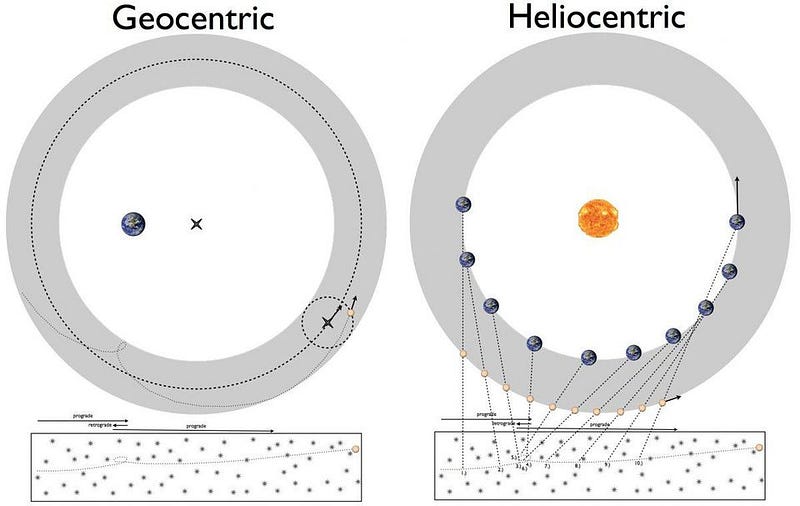
Let’s consider the following statement: “the Earth is round.” If you’re not a scientist (and also not a flat-Earther), you might think that this statement is unimpeachable. You might think of this as being scientifically true. In fact, stating that the Earth is round is a valid scientific conclusion and a scientific fact, at least if you contrast a round Earth with a flat Earth.
But there’s always an additional nuance and caveat at play. If you were to measure the diameter of the Earth across our equator, you’d get a value: 7,926 miles (12,756 km). If you measured the diameter from the north pole to the south pole, you’d get a slightly different value: 7,900 miles (12,712 km). The Earth is not a perfect sphere, but rather a near-spherical shape that bulges at the equator and is compressed at the poles.
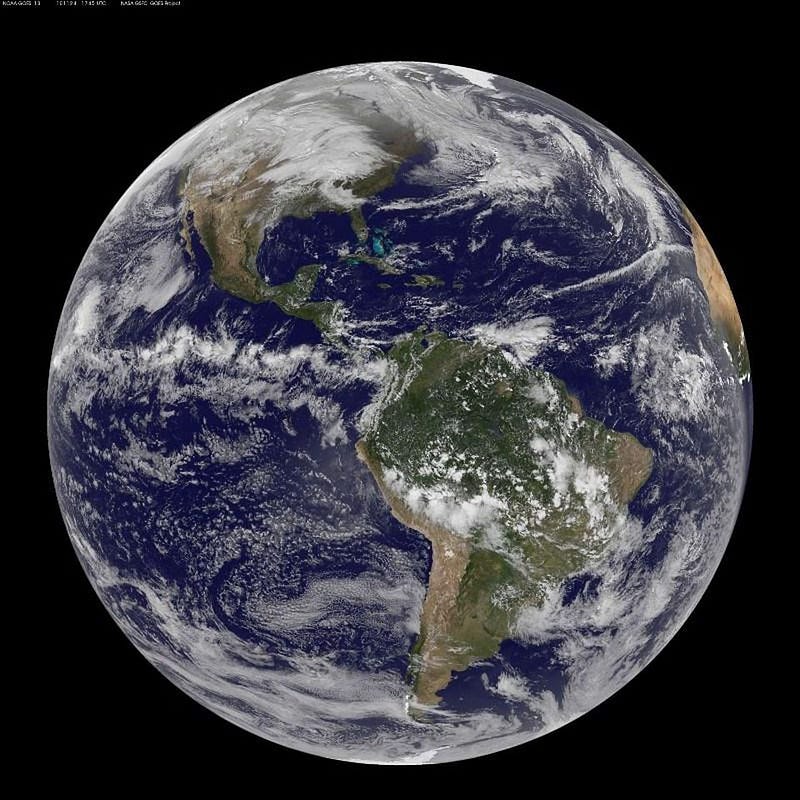
To a scientist, this illustrates extremely well the caveats associated with a term like scientific truth. Sure, it’s more true that the Earth is a sphere than that the Earth is a disc or a circle. But it isn’t an absolute truth that the Earth is a sphere, because it’s more correct to call it an oblate spheroid than a sphere. And even if you do, calling it an oblate spheroid isn’t the absolute truth, either.
There are surface features on Earth that demonstrate significant departures from a smooth shape like either a sphere or an oblate spheroid. There are mountain ranges, rivers, valleys, plateaus, deep oceans, trenches, ridges, volcanoes and more. There are locations where the land extends more than 29,000 feet (nearly 9,000 meters) above sea level, and places where you won’t touch the Earth’s surface until you’re 36,000 feet (11,000 meters) beneath the ocean’s surface.
This example highlights a few important ways of thinking scientifically that differ from how we think colloquially.
- There are no absolute truths in science; there are only approximate truths.
- Whether a statement, theory, or framework is true or not depends on quantitative factors and how closely you examine or measure the results.
- Every scientific theory has a finite range of validity: inside that range, the theory is indistinguishable from true, outside of that range, the theory is no longer true.
This represents an enormous difference from how we commonly think about fact vs. fiction, truth vs. falsehood, or even right vs. wrong.
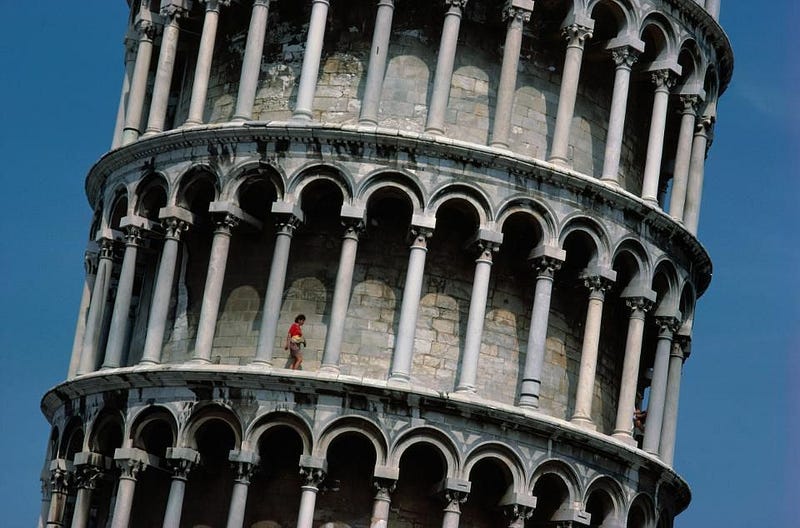
For example, if you drop a ball on Earth, you can ask the quantitative, scientific question of how it will behave. Like everything on Earth’s surface, it will accelerate downwards at 9.8 m/s² (32 ft/s²). And this is a great answer, because it’s approximately true.
In science, though, you can start looking more deeply, and seeing where this approximation is no longer true. If you perform this experiment at sea level, at a variety of latitudes, you’ll find that this answer actually varies: from 9.79 m/s² at the equator to 9.83 m/s² at the poles. If you go to higher altitudes, you’ll find that the acceleration starts to slowly decrease. And if you leave the Earth’s gravitational pull, you’ll find that this rule isn’t universal at all, but is rather superseded by a more general rule: the law of Universal gravitation.
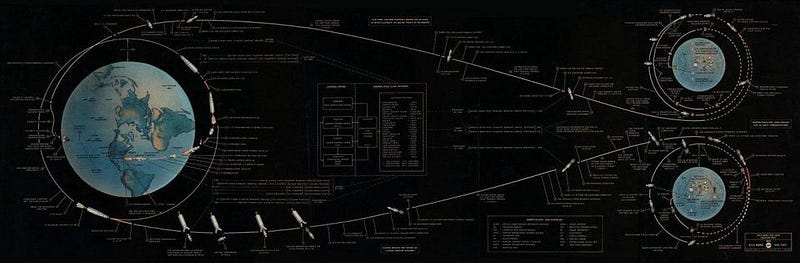
This laws is even more generally true. Newton’s law of universal gravitation can explain all the successes of modeling Earth’s acceleration as a constant, but it can also do much more. It can describe the orbital motion of the moons, planets, asteroids and comets of the solar system, as well as how much you’d weigh on any of the planets. It describes how the stars move around inside galaxies, and even allowed us to predict how to send a rocket to land humans on the Moon, with extraordinarily accurate trajectories.
But even Newton’s law has its limits. When you move close to the speed of light, or get very close to an extremely large mass, or want to know what’s occurring on cosmic scales (such as in the case of the expanding Universe), Newton won’t help you. For that, you have to supersede Newton and move on to Einstein’s General Relativity.
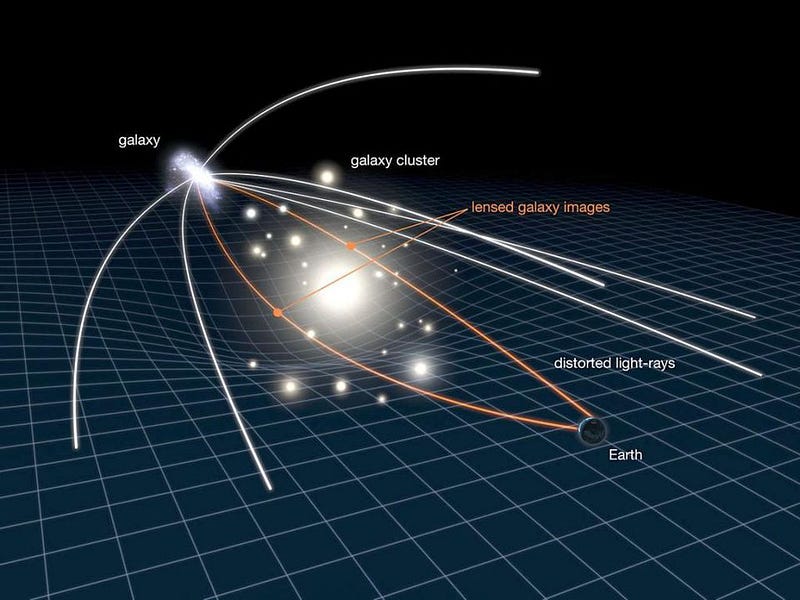
For the trajectories of particles moving close to the speed of light, or to obtain very accurate predictions for the orbit of Mercury (the Solar System’s closest and fastest planet), or to explain the gravitational bending of starlight by the Sun (during an eclipse) or by a large collection of mass (such as in the case of gravitational lensing, above), Einstein’s theory gets it right where Newton’s fails. In fact, for every observational or experimental test we’ve thrown at General Relativity, from gravitational waves to the frame-dragging of space itself, it’s passed with flying colors.
Does that mean that Einstein’s theory of General Relativity can be taken as a scientific truth?
When you apply it to these specific scenarios, absolutely. But there are other scenarios we can apply it to, all of which are not yet sufficiently tested, where we fully expect that it won’t give quantitatively accurate predictions.
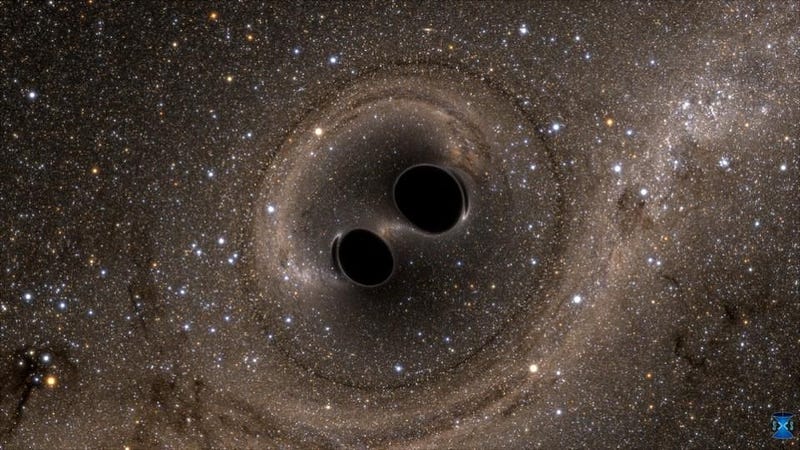
There are many questions we can ask about reality that require us to understand what’s happening where gravity is important or where the curvature of spacetime is extremely strong: just where you’d want Einstein’s theory. But when the distance scales you’re thinking about are also very small, you expect quantum effects to be important as well, and General Relativity cannot account for those. These include questions such as the following:
- What happens to the gravitational field of an electron when it passes through a double slit?
- What happens to the information of the particles that form a black hole, if the black hole’s eventual state is to decay into thermal radiation?
- And what is the behavior of a gravitational field/force at and around a singularity?
Einstein’s theory won’t just get these answers wrong, it won’t have sensible answers to offer. In these regimes, we know we require a more advanced theory, such as a valid quantum gravitational theory, to tell us what’s going to happen under these circumstances.
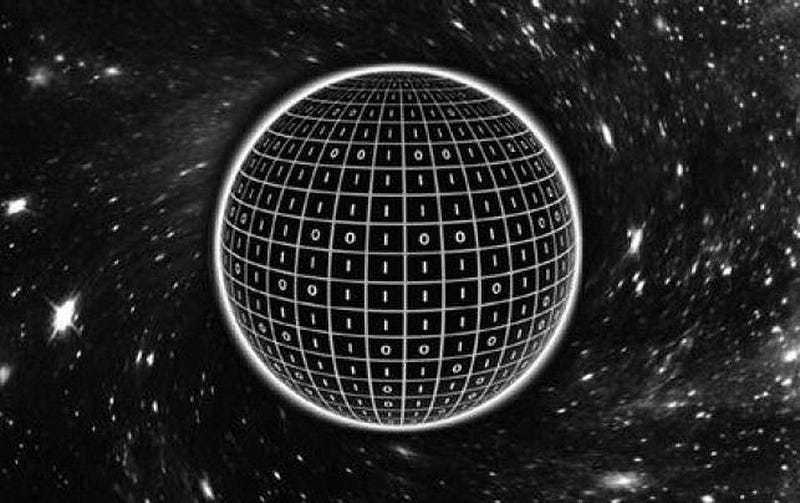
Yes, masses at the surface of Earth accelerate downwards at 9.8 m/s², but if we ask the right questions or perform the right observations or experiments, we can find where and how this description of reality is no longer a good approximation of the truth. Newton’s laws can explain that phenomenon and many others, but we can find observations and experiments that show us where Newton, too, is insufficient.
Even replacing Newton’s laws with Einstein’s General Relativity leads to the same story: Einstein’s theory can successfully explain everything that Newton’s can, plus additional phenomena. Some of those phenomena were already known when Einstein was constructing his theory; others had not yet been tested. But we can be certain that even Einstein’s greatest accomplishment will someday be superseded. When it does, we fully expect it will happen in exactly the same way.

Science is not about finding the absolute truth of the Universe. No matter how much we’d like to know what the fundamental nature of reality is, from the smallest subatomic scales to the largest cosmic ones and beyond, this is not something science can deliver. All of our scientific truths are provisional, and we must recognize that they are only models or approximation of reality.
Even the most successful scientific theories imaginable will, by their very nature, have a limited range of validity. But we can theorize whatever we like, and when a new theory meets the following three criteria:
- it achieves all of the successes of the prevailing, pre-existing theory,
- it succeeds where the current theory is known to fail,
- and it makes novel predictions for hitherto unmeasured phenomena, distinct from the prior theory, that pass the critical observational or experimental tests,
it will supersede the current one as our best approximation of a scientific truth.
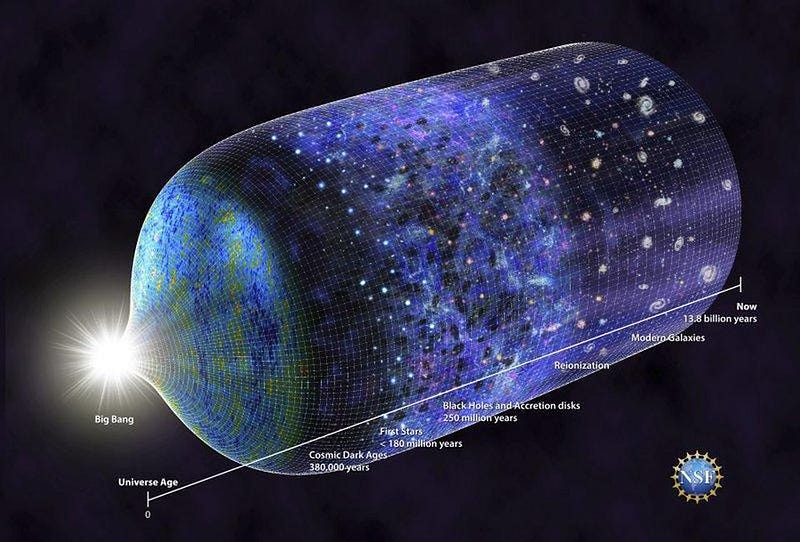
All of our currently held scientific truths, from the Standard Model of elementary particles to the Big Bang to dark matter and dark energy to cosmic inflation and beyond, are only provisional. They describe the Universe extremely accurately, succeeding in regimes where all prior frameworks have failed. Yet they all have limitations to how far we can take their implications before we arrive at a place where their predictions are no longer sensible, or no longer describe reality. They are not absolute truths, but approximate, provisional ones.
No experiment can ever prove that a scientific theory is true; we can only demonstrate that its validity either extends or fails to extend to whatever regime we test it in. The failure of a theory is actually the ultimate scientific success: an opportunity to find an even better scientific truth to approximate reality. It’s being wrong in the best way imaginable.
Send in your Ask Ethan questions to startswithabang at gmail dot com!
Ethan Siegel is the author of Beyond the Galaxy and Treknology. You can pre-order his third book, currently in development: the Encyclopaedia Cosmologica.