Five Discoveries In Fundamental Physics That Came As Total Surprises
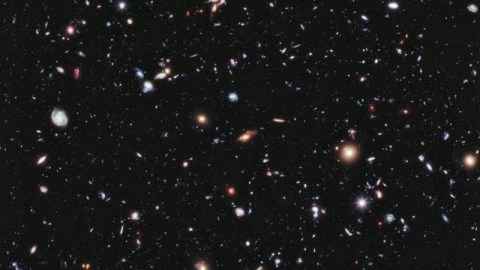
If you think we know it all, you’ll never be ready for the next big breakthrough.
When you’re taught the scientific method, you think of a neat procedure you can follow to gain insight into some natural phenomenon about the Universe. Start with an idea, perform an experiment, and either validate or falsify the idea, depending on the outcome. Only, the real world is a lot messier than that. Sometimes, you perform an experiment, and you get a result that’s entirely different from what you were expecting. And sometimes, the correct explanation requires a leap of imagination that goes far beyond what any reasonable person would logically conclude. Today, the physical Universe is very well understood, but the story of how we got here is full of surprises. If we want to progress further, there are likely even more in store. Here are a look back at five of the greatest ones in history.
1.) The speed of light doesn’t change when you boost your light source. Imagine throwing a ball as fast as you can. Depending on what sport you’re playing, you might get all the way up to 100 miles per hour (~45 meters/second) using your hand-and-arm alone. Now, imagine you’re on a train (or in a plane) moving incredibly quickly: 300 miles per hour (~134 m/s). If you throw the ball from the train, moving in the same direction, how fast does the ball move? You simply add the speeds up: 400 miles per hour, and that’s your answer. Now, imagine that instead of throwing a ball, you emit a beam of light instead. Add the speed of the light to the speed of the train… and you get an answer that’s completely wrong.
Really, you do! This was the central idea of Einstein’s theory of special relativity, but it wasn’t Einstein who made this experimental discovery; it was Albert Michelson, who’s pioneering work in the 1880s demonstrated that this was the case. Whether you fired a beam of light in the same direction that Earth moved, perpendicular to that direction, or antiparallel to that direction made no difference. Light always moved at the same speed: c, the speed of light in vacuum. Michelson developed his interferometer to measure the motion of the Earth through the aether, and instead paved the way for relativity. His 1907 Nobel prize remains the world’s most famous null result, and the most important one in scientific history.
2.) 99.9% of an atom’s mass is concentrated in an incredibly dense nucleus. Have you ever heard of the ‘plum pudding’ model of the atom? It sounds quaint today, but it was generally accepted at the start of the 20th century that atoms were made of a mix of negatively charged electrons (behaving like plums) embedded in a positively-charged medium (which behaved like pudding) that filled all of space. Electrons could be stripped off or stolen, explaining the phenomenon of static electricity. For years, J.J. Thomson’s model of a composite atom, with small electrons in a positively charged substrate, was generally accepted. Until, that is, it was put to the test by Ernest Rutherford.
By firing high-energy, charged particles (from radioactive decays) at a very thin sheet of gold foil, Rutherford fully expected that all the particles would pass through. And most of them did, but a few spectacularly bounced back! As Rutherford recounted:
It was quite the most incredible event that has ever happened to me in my life. It was almost as incredible as if you fired a 15-inch shell at a piece of tissue paper and it came back and hit you.
What Rutherford discovered was the atomic nucleus, containing virtually all the mass of an atom, confined to a volume one-quadrillionth (10–15) the size of the entire thing. It was the birth of modern physics, and it paved the way for the quantum revolution of the 20th century.
3.) ‘Missing energy’ leads to the discovery of a minuscule, nearly-invisible particle. In all the interactions we’ve ever seen between particles, energy is always conserved. It can be transformed from one type into another — potential, kinetic, rest mass, chemical, atomic, electrical, etc. — but it can never be created nor destroyed. Which is why it was so puzzling, nearly a century ago, when it was found that some radioactive decays have slightly less total energy in their products than in the initial reactants. It led Bohr to postulate that energy was always conserved… except for when it was lost. But Bohr was mistaken, and it was Pauli who had other ideas.
Pauli contended that energy must be conserved, and so way back in 1930, he proposed a new particle: the neutrino. This “little neutral one” would not interact electromagnetically, but would instead have a minuscule mass and carry kinetic energy away. While many were skeptical, experiments from the products of nuclear reactions eventually detected both neutrinos and antineutrinos in the 1950s and 1960s, which helped lead physicists to both the Standard Model and the model of the weak nuclear interactions. It’s a stunning example of how theoretical predictions can sometimes lead to a spectacular advance, once the proper experimental techniques are developed.
4.) All the particles we interact with have high-energy, unstable cousins. It’s often said that advances in science aren’t met with “eureka!” but rather with “that’s funny,” but this actually happened in fundamental physics! If you charge up an electroscope — where two conducting metal leaves are connected to another conductor — both leaves will gain the same electric charge, and repel one another as a result. If you place that electroscope in a vacuum, the leaves shouldn’t discharge, but over time, they do. The best idea we had for this discharge was that there were high-energy particles hitting Earth from outer space, cosmic rays, and the products of these collisions were discharging the electroscope.
In 1912, Victor Hess conducted balloon-borne experiments to search for these high-energy cosmic particles, discovering them immediately in great abundance and becoming the father of cosmic rays. By constructing a detection chamber with a magnetic field in them, you could measure both the velocity and charge-to-mass ratio based on how the particle’s track curves. Protons, electrons, and even the first particles of antimatter were detected via this method, but the biggest surprise came in 1933, when Paul Kunze, working with cosmic rays, discovered a track from a particle that was just like the electron… except hundreds of times heavier!
The muon, with a lifetime of just 2.2 microseconds, was later experimentally confirmed and detected by Carl Anderson and his student, Seth Neddermeyer, using a cloud chamber on the ground. When the physicist I.I. Rabi, himself a Nobel Laureate for the discovery of nuclear magnetic resonance, learned of the muon’s existence, he famously quipped, “Who ordered that?” It was later discovered that both composite particles (like the proton and neutron) and fundamental ones (quarks, electrons, and neutrinos) all have multiple generations of heavier relatives, with the muon being the first “generation 2” particle ever discovered.
5.) The Universe began with a bang, but that discovery was a complete accident. In the 1940s, George Gamow and his collaborators put forth a radical idea: that the Universe that was expanding and cooling today was not only hotter and denser in the past, but arbitrarily so. If you extrapolated back far enough, you’d have a Universe hot enough to ionize all the matter in it, while even farther back you’d break apart atomic nuclei. The idea became known as the Big Bang, with two major predictions arising:
- The Universe we began with wouldn’t have only matter made of mere protons and electrons, but would consist of a mix of the light elements, fused together in the high-energy, early Universe.
- When the Universe cooled enough to form neutral atoms, that high-energy radiation would be released, and would travel in a straight line for all eternity until it collided with something, redshifting and losing energy as the Universe expanded.
This “cosmic microwave background” was predicted to be just a few degrees above absolute zero.
In 1964, Arno Penzias and Bob Wilson accidentally discovered the Big Bang’s leftover glow. Working with a radio antenna at Bell Labs to study radar, they found uniform noise everywhere they looked on the sky. It wasn’t the Sun, or the galaxy, or Earth’s atmosphere… but they didn’t know what it was. So they cleaned out the inside of the antenna with mops, removing pigeons in the process, but still the noise persisted. It was only when the results were shown to a physicist familiar with the Princeton group’s (Dicke, Peebles, Wilkinson, etc.) detailed predictions, and with the radiometer they were building to detect exactly this type of signal, that they recognized the significance of what they found. For the first time, the origin of our Universe was known.
When we look back at the body of scientific knowledge we have today, at its predictive power and how the centuries of discoveries have transformed our lives, it’s tempting to view science as a steady progression of ideas. But in reality, the history of science is messy, full of surprises, and laden with controversy. For those working on the cutting edge at the time, science involves taking risks, exploring novel scenarios, and striking out in a direction never attempted before. While the history we recount is filled with the success stories, real history is fraught with blind alleys, failed experiments, and outright mistakes. Nevertheless, an open mind, a willingness and ability to test your ideas, and our capacity to learn from our results and revise our conclusions leads us out of the darkness and into the light. At the end of the day, we all win.
Ethan Siegel is the author of Beyond the Galaxy and Treknology. You can pre-order his third book, currently in development: the Encyclopaedia Cosmologica.